You are using an outdated browser. Please upgrade your browser or activate Google Chrome Frame to improve your experience.
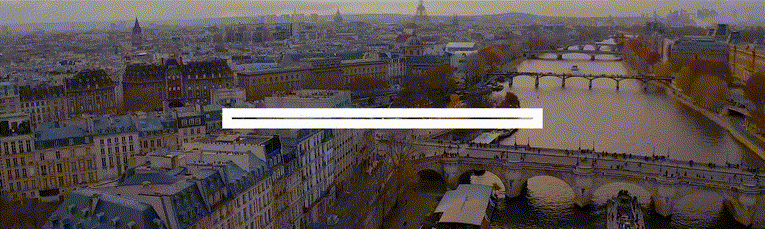
Western flower thrips
Frankliniella occidentalis (Pergande) (Thysanoptera: Thripidae) originated in western North America and has since become a major pest of vegetables, fruit and ornamental crops across the US and around the world. F. occidentalis are small (1-2 mm long), slender, soft-bodied insects that are yellow to light brown in color; adults have distinctive fringed wings. It can develop quickly, going from egg to adult in two weeks or less at favorable temperatures. Adult females insert eggs into plant tissue under the epidermis. When mature, larvae drop to the soil to go through the prepupal and pupal stages, and finally return to the plants as adults. Larvae and adults feed on flowers, buds, terminals, leaves, and fruit.
Frankliniella occidentalis feed by rasping open plant cells and sucking up the cell contents. The damaged cells collapse, leaving bronzed or russeted areas on the leaves or fruits. Besides the direct plant damage this pest causes, F. occidentalis also transmits several species of destructive plant viruses in the genus Tospovirus (Bunyaviridae), including Tomato Spotted Wilt Virus (TSWV) and Impatiens Necrotic Spot Virus (INSV), of which it is the most important vector worldwide.
Adults can move long distances on air currents to find new food; adults and larvae can also be transported on transplants. Although there are some effective natural enemies of F. occidentalis , growers rely on chemical control to reduce damaging populations of this pest. Natural enemies such as predatory bugs ( Orius spp.), lacewings ( Chrysoperla spp.) and predatory mites ( Amblyseius spp., Neoseiulus spp.) can provide significant control of F. occidentalis populations. The number of effective chemical compounds that control F. occidentalis is very limited and insecticide resistance has been reported to several major classes of insecticides.
Western flower thrips resistance profile
Susceptibility test methods.
- IRAC Susceptibility Test Method 014 Larvae Dip
- IRAC Susceptibility Test Method 010 Adults Dip Feature video
External links
- Bayer Crop Compendium - Frankliniella occidentalis
- Plantwise Knowledge Bank - Frankliniella occidentalis
Key western flower thrips resources
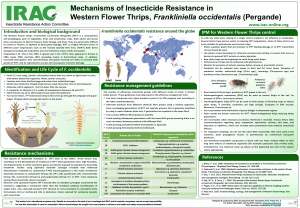
The information provided is based on literature reviews and as such IRAC cannot guarantee or be held accountable for the accuracy of the reports.
We use anonymous data in cookies to understand website usage. You consent to our cookies if you continue to use this website.
- Research article
- Open access
- Published: 19 October 2020
Genome-enabled insights into the biology of thrips as crop pests
- Dorith Rotenberg ORCID: orcid.org/0000-0002-9018-8822 1 ,
- Aaron A. Baumann 2 ,
- Sulley Ben-Mahmoud 3 ,
- Olivier Christiaens 4 ,
- Wannes Dermauw 4 ,
- Panagiotis Ioannidis 5 , 6 ,
- Chris G. C. Jacobs 7 ,
- Iris M. Vargas Jentzsch 8 ,
- Jonathan E. Oliver 9 ,
- Monica F. Poelchau 10 ,
- Swapna Priya Rajarapu 1 ,
- Derek J. Schneweis 11 ,
- Simon Snoeck 12 , 4 ,
- Clauvis N. T. Taning 4 ,
- Dong Wei 4 , 13 , 14 ,
- Shirani M. K. Widana Gamage 15 ,
- Daniel S. T. Hughes 16 ,
- Shwetha C. Murali 16 ,
- Samuel T. Bailey 17 ,
- Nicolas E. Bejerman 18 ,
- Christopher J. Holmes 17 ,
- Emily C. Jennings 17 ,
- Andrew J. Rosendale 17 , 19 ,
- Andrew Rosselot 17 ,
- Kaylee Hervey 11 ,
- Brandi A. Schneweis 11 ,
- Sammy Cheng 20 ,
- Christopher Childers 10 ,
- Felipe A. Simão 6 ,
- Ralf G. Dietzgen 21 ,
- Hsu Chao 16 ,
- Huyen Dinh 16 ,
- Harsha Vardhan Doddapaneni 16 ,
- Shannon Dugan 16 ,
- Yi Han 16 ,
- Sandra L. Lee 16 ,
- Donna M. Muzny 16 ,
- Jiaxin Qu 16 ,
- Kim C. Worley 16 ,
- Joshua B. Benoit 17 ,
- Markus Friedrich 22 ,
- Jeffery W. Jones 22 ,
- Kristen A. Panfilio 8 , 23 ,
- Yoonseong Park 24 ,
- Hugh M. Robertson 25 ,
- Guy Smagghe 4 , 13 , 14 ,
- Diane E. Ullman 3 ,
- Maurijn van der Zee 7 ,
- Thomas Van Leeuwen 4 ,
- Jan A. Veenstra 26 ,
- Robert M. Waterhouse 27 ,
- Matthew T. Weirauch 28 , 29 ,
- John H. Werren 20 ,
- Anna E. Whitfield 1 ,
- Evgeny M. Zdobnov 6 ,
- Richard A. Gibbs 16 &
- Stephen Richards 16
BMC Biology volume 18 , Article number: 142 ( 2020 ) Cite this article
9664 Accesses
46 Citations
120 Altmetric
Metrics details
An Author Correction to this article was published on 16 November 2020
This article has been updated
The western flower thrips, Frankliniella occidentalis (Pergande), is a globally invasive pest and plant virus vector on a wide array of food, fiber, and ornamental crops. The underlying genetic mechanisms of the processes governing thrips pest and vector biology, feeding behaviors, ecology, and insecticide resistance are largely unknown. To address this gap, we present the F. occidentalis draft genome assembly and official gene set.
We report on the first genome sequence for any member of the insect order Thysanoptera. Benchmarking Universal Single-Copy Ortholog (BUSCO) assessments of the genome assembly (size = 415.8 Mb, scaffold N50 = 948.9 kb) revealed a relatively complete and well-annotated assembly in comparison to other insect genomes. The genome is unusually GC-rich (50%) compared to other insect genomes to date. The official gene set (OGS v1.0) contains 16,859 genes, of which ~ 10% were manually verified and corrected by our consortium. We focused on manual annotation, phylogenetic, and expression evidence analyses for gene sets centered on primary themes in the life histories and activities of plant-colonizing insects. Highlights include the following: (1) divergent clades and large expansions in genes associated with environmental sensing (chemosensory receptors) and detoxification (CYP4, CYP6, and CCE enzymes) of substances encountered in agricultural environments; (2) a comprehensive set of salivary gland genes supported by enriched expression; (3) apparent absence of members of the IMD innate immune defense pathway; and (4) developmental- and sex-specific expression analyses of genes associated with progression from larvae to adulthood through neometaboly, a distinct form of maturation differing from either incomplete or complete metamorphosis in the Insecta.
Conclusions
Analysis of the F. occidentalis genome offers insights into the polyphagous behavior of this insect pest that finds, colonizes, and survives on a widely diverse array of plants. The genomic resources presented here enable a more complete analysis of insect evolution and biology, providing a missing taxon for contemporary insect genomics-based analyses. Our study also offers a genomic benchmark for molecular and evolutionary investigations of other Thysanoptera species.
Thrips are small, polyphagous, and cosmopolitan insects that comprise the order Thysanoptera. Thysanoptera lies within the Paraneoptera, also commonly called the “hemipteroid assemblage” which also includes the orders Hemiptera, Psocoptera, and Phthiraptera. Among the over 7000 reported thrips species classified into nine families with an additional five identified from fossil species [ 1 ], the plant-feeders and crop pests are the most well-characterized members of the order due to their agricultural importance. Thysanopterans present a diverse array of biological, structural, and behavioral attributes, but share characteristics that are unique to insects in the order. Among these are fringed wings (Fig. 1 a, Adult panel) and a complex mouthcone (Fig. 1 b, c) that houses asymmetrical mouthparts composed of three stylets (Fig. 1 d). The paired, maxillary stylets interlock when extended during ingestion, forming a single tube, i.e., food canal, that is also thought to serve as a conduit for saliva, while the single, solid-ended mandibular stylet (peg) is used to pierce substrates (its counterpart is resorbed during embryonic development) [ 6 , 7 ]. All the stylets are innervated, giving thrips control of stylet direction and movement in response to sensory cues [ 8 ]. Thrips also have mechano- and chemosensory structures likely governing host finding and choice. The external surface of the mouthcone supports 10 sensory pegs on each paraglossa, nine of which appear to have a dual chemosensory and mechanosensory function (sensory pegs 1–5, 7–10), and one with a mechanosensory function (sensory peg 6) (Fig. 1 e). In addition, internally, there are precibarial and cibarial chemosensory structures, likely important in feeding choices [ 8 ].
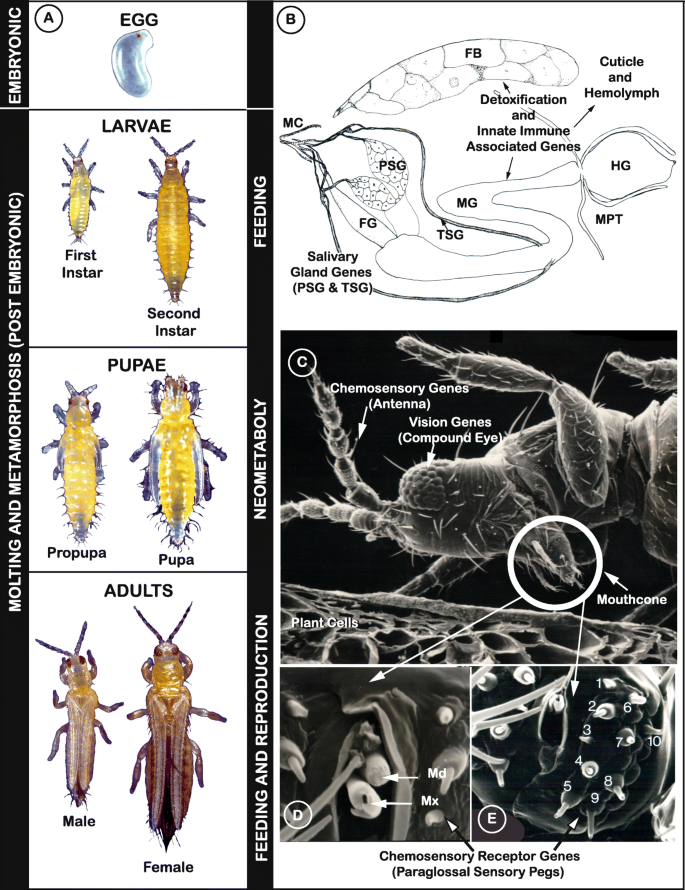
Illustration of how curated gene sets intertwine with understanding of biological processes of Frankliniella occidentalis . a Developmental stages. Vertical bars: (left) embryonic and postembryonic stages associated with developmental and sex-specific expression analyses of genes underlying molting and metamorphosis through neometaboly; (right) larval and adult stages feed and are associated with divergent clades and expansions in gene families related to host selection and feeding (vision, chemosensation) and detoxification of xenobiotics; propupal and pupal stages do not feed; adults reproduce by arrhenotokous parthenogenesis. Modified from [ 2 ], permission of CAB International through PLSclear. b Cartoon depicting principal and tubular salivary glands (PSG, TSG) associated with enriched expression of specific genes, and the midgut (MG), hindgut (HG), Malpighian tubules (MPT), and fat body (FB), important sites for detoxification and innate immunity gene sets along with the hemolymph and cuticle. Modified from [ 3 ], permission by Elsevier. c Scanning electron micrograph (SEM) of adult pre-probing behavior highlights compound eyes used in visual aspects of host finding (associated with opsin genes); external antennal and mouthcone sensory structures essential to host finding, choice, and feeding; likely associated with expanded gene families underlying chemosensation. Internal leaf anatomy shows cells most commonly fed on. Modified from [ 4 ], permission from Springer-Verlag. d SEM showing the tips of the single mandible (Md) and paired maxillae (Mx) forming the feeding tube. Modified from [ 5 ], permission of Elsevier. e Mouthcone paraglossal sensory pegs (numbered, left paraglossa)—pegs 1–5, 7–10, are dual function (mechano- and chemosensory), peg 6 is mechanosensory; their location suggests importance in detecting plant surface microtopography and chemistry during host and feeding-site selection and association with divergent and expanded gene families related to environmental sensing. Modified from [ 5 ], permission of Elsevier
Also remarkable is their postembryonic development, referred to as “remetaboly” [ 9 ] and more recently termed “neometabolous” [ 10 ] (Fig. 1 a). This developmental strategy has been described as intermediate between holo- and hemimetabolous because the two immobile and non-feeding pupal stages (propupae and/or pupae) (Fig. 1 a, Pupae panel) undergo significant histolysis and histogenesis, yet the emergent adult body plan largely resembles that of the larva except for the presence of wings and mature reproductive organs (Fig. 1 a, Adult panel).
Frankliniella occidentalis (suborder Terebrantia, family Thripidae, subfamily Thripinae) is a devastatingly invasive crop pest species with a global geographical distribution and an extraordinarily broad host range, capable of feeding on hundreds of diverse plant species, tissue types, fungi, and other arthropods. Additionally, this species has developed resistance to diverse insecticides with varying modes of action [ 11 , 12 , 13 ]. For example, on cotton, there have been 127 cases reported of field-evolved resistance to 19 insecticides belonging to six groups (modes of action) of insecticides [ 14 ]. The insect is haplo-diploid, i.e., haploid males arise from unfertilized eggs, while diploid females develop from fertilized eggs [ 15 ]. The short reproductive cycle and high fecundity of this species contributes to its success as an invasive species.
In addition to direct damage to plants, F. occidentalis and other thrips vectors interact with and transmit diverse types of plant pathogens [ 16 , 17 , 18 , 19 ], most notoriously orthotospoviruses [ 20 , 21 , 22 ], to a wide array of food, fiber, and ornamental crops around the globe. With regard to orthotospovirus-thrips interactions, global expression analyses of whole bodies of F. occidentalis [ 23 , 24 ] and other thrips vectors [ 25 , 26 ] indicated the occurrence of insect innate immune responses to virus infection. In addition to serving as crop disease vectors, thrips support facultative bacterial symbionts that reside in the hindgut [ 27 , 28 ].
While there are numerous studies centered on thrips systematics, feeding behaviors, ecology, virus transmission biology, pest biology and insecticide resistance [ 29 ], the underlying genetic mechanisms of the complex and dynamic processes governing these areas of research are largely unknown. Here we present the F. occidentalis genome assembly and annotation, with phylogenetic analyses and genome-referenced transcriptome-wide expression data of gene sets centered on primary themes in the life histories and activities of plant-colonizing insects: (1) host-locating and chemical sensory perception (Fig. 1 c–e), (2) plant feeding and detoxification (Fig. 1 b, c), (3) innate immunity (Fig. 1 b), and (4) development and reproduction (Fig. 1 a). Analysis of the F. occidentalis genome highlights evolutionary divergence and host adaptations of plant-feeding thysanopterans compared to other taxa. Our findings underscore the ability of F. occidentalis to sense diverse food sources, to feed on and detoxify an array of natural compounds (e.g., plant secondary compounds) and agrochemicals (e.g., insecticides), and to combat and/or support persistent microbial associations. We also provide insights into thrips development and reproduction. This is the first thysanopteran genome to be sequenced, and the annotations and resources presented herein provide a platform for further analysis and better understanding of not just F. occidentalis , but all members of this intriguing insect order.
Results and discussion
Genome metrics.
The assembly size of the F. occidentalis draft genome (Focc_1.0) was determined to be 416 Mb (Table 1 ), including gaps, which is larger than the published genome size estimate obtained by flow cytometry of propidium iodide-stained nuclei of adult males (337.4 ± 4.3 Mb) and females (345 ± 5 Mb) of F. occidentalis (see Table 1 in [ 31 ]). The assembly consists of 6263 scaffolds (N50 = 948 kb). One striking feature of the genome is the GC content of ~ 50%, extraordinarily larger than other insects to date [ 32 ]. Updated assemblies with reduced proportions of gaps yielded total assembly sizes of 275–278 Mbp (see “ Methods ”); however, already accumulated manual annotations could not be comprehensively mapped to these new assemblies so the community reverted to using the original assembly.
Phylogenomics with a complete and well-annotated genome assembly
Phylogenomic analysis correctly placed F. occidentalis (Insecta: Thysanoptera) basal to Acyrthosiphon pisum and Cimex lectularius (Insecta: Hemiptera) (Fig. 2 a). Unexpectedly, however, the body louse Pediculus humanus (Insecta: Psocodea) appears as an outgroup to all other insects, which disagrees with previous findings [ 33 ]. This discordance is most likely due to taxon sampling and would likely be resolved when more genome sequences become available from early-diverging insect lineages (e.g., Paleoptera). BUSCO assessments (see “ Methods ”) showed that both the genome assembly (Fig. 2 b, left bars, C:99.0%, S:97.6%, D:1.4%, F:0.5%, M:0.6% n:1066) and the official gene set (OGS) (Fig. 2 b, right bars, C:99.1%, S:97.6%, D:1.5%, F:0.6%, M:0.4% n:1066) of F. occidentalis are very complete when compared to those of other arthropods. Moreover, the OGS-based BUSCO scores are slightly better than the genome-based scores, resulting in reduced numbers of missing BUSCOs. These findings indicate that the F. occidentalis gene annotation strategy successfully managed to capture even difficult-to-annotate genes.
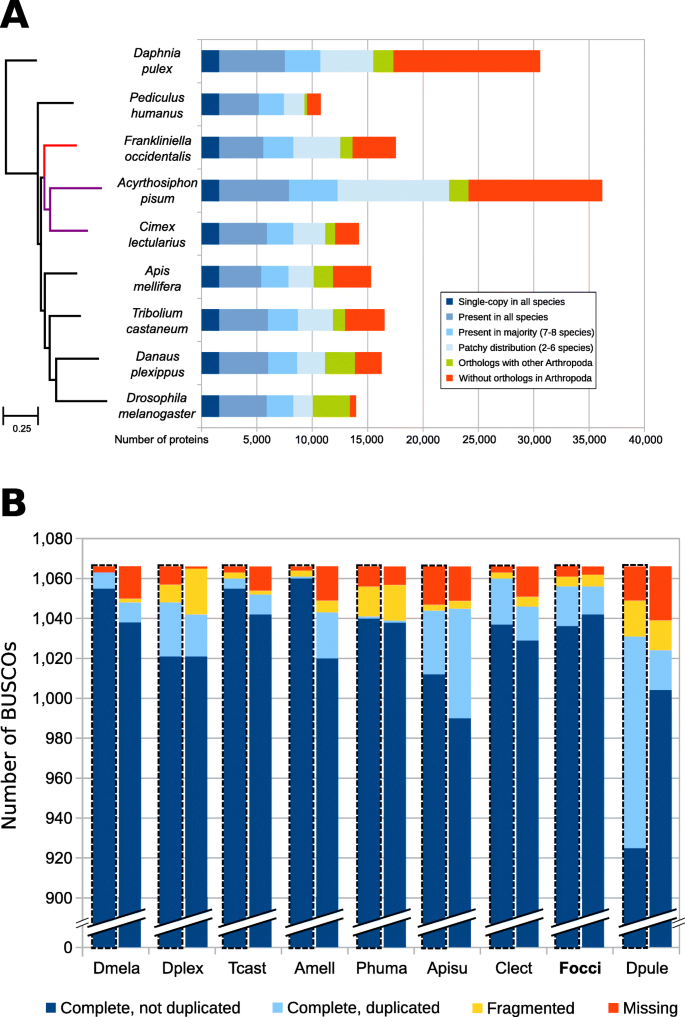
Phylogeny and orthology of Frankliniella occidentalis with other arthropods, with genome and gene set completeness assessments. a The phylogenomic analysis was based on the aligned amino acid sequences of 1604 single-copy orthologs and placed F. occidentalis (shown in red) as basal to the hemipteran species Acyrthosiphon pisum and Cimex lectularius (shown in purple). All nodes have bootstrap support of 100% and the scale bar corresponds to substitutions per site. OrthoDB orthology delineation with the protein-coding genes from the F. occidentalis official gene set identify genes with orthologs in all or most of the representative insects and the outgroup species, Daphnia pulex , as well as those with more limited distributions or with no confidently identifiable arthropod orthologs. b Assessments using the 1066 arthropod Benchmarking Universal Single-Copy Orthologs (BUSCOs) show few missing genes (5 for the assembly, 4 for the OGS) from F. occidentalis , with better OGS completeness than A. pisum , C. lectularius , and P. humanus . The F. occidentalis official gene set (OGS) scores better than its genome assembly, indicating that the gene annotation strategy has successfully managed to capture even difficult to annotate genes. The left bars for each species, also outlined with a dashed line, show the results based on the genome, whereas the right bars show the results for the OGSs. Species names abbreviations: Dmela— Drosophila melanogaster , Dplex— Danaus plexippus , Tcast— Tribolium castaneum , Amell— Apis mellifera , Phuma— Pediculus humanus , Apisu— Acyrthosiphon pisum , Clect— Cimex lectularius , Focci— Frankliniella occidentalis , Dpule— Daphnia pulex
Assembly quality assessment via Hox gene copy number and cluster synteny
The Hox and Iro-C gene clusters that encode homeodomain transcription factors are highly conserved in bilaterian animals and in insects, respectively [ 34 , 35 , 36 ], and offer an additional quality appraisal for genome assembly. All single-copy gene models for the expected Hox and Iro-C orthologs were successfully constructed (Additional file 1 : Section 1, Table S1.1), and with regard to synteny, we could reconstitute the small Iro-C cluster and partially assemble the larger Hox cluster (Additional file 1 : Section 1, Figure S1.1), with linkage for Hox2/3/4 , Hox5/6/7 , and Hox8/9/10 . All linked Hox genes occurred in the expected order and with the expected, shared transcriptional orientation, albeit with some missing coding sequence for some gene models. However, direct concatenation of the four scaffolds with Hox genes would yield a Hox cluster of 5.9 Mb in a genome assembly of 416 Mb, which is disproportionately large (3.5-fold larger relative cluster size compared to the beetle Tribolium castaneum and other, de novo insect genomes [ 37 , 38 , 39 , 40 ]).
Interestingly, although orthology is clear for all ten Hox genes, they are rather divergent compared to other insects. Specifically, several F. occidentalis Hox genes have acquired novel introns in what are generally highly conserved gene structures, and several Hox genes encode unusually large proteins compared to their orthologs, corroborating a previous, pilot analysis on unique protein-coding gene properties in this unusually GC-rich genome ([ 38 ], see supplement). Global comparisons of structural properties with other insects further confirm that the F. occidentalis genome is unusual for the combination of high GC content, large protein sizes, and short exons [ 41 ]. It will be interesting to see whether these trends are borne out as genome data become available for more Thysanoptera.
Genome-wide analysis of transcription factors
In addition to the selected homeodomain proteins, we comprehensively identified likely transcription factors (TFs) among our entire OGS by scanning the amino acid sequences of predicted protein-coding genes for putative DNA-binding domains (DBDs). When possible, we also predicted the DNA-binding specificity of each TF. Using this approach, we discovered 843 putative TFs in the F. occidentalis genome, which is similar to other insect genomes (e.g., 701 for Drosophila melanogaster ). Likewise, the number of members of each F. occidentalis TF family is comparable to that of other insects (Fig. 3 a). Of the 843 F. occidentalis TFs, we were able to infer motifs for 197 (23%) (Additional file 2 : Table S5), mostly based on DNA-binding specificity data from D. melanogaster (120 TFs), but also from species as distant as human (43 TFs) and mouse (12 TFs). Many of the largest TF families have inferred motifs for a substantial proportion of their TFs, including homeodomain/Hox (64 of 78, 82%), bHLH (30 of 36, 83%), and nuclear receptors (11 of 17, 65%). As expected, the largest gap is for C 2 H 2 zinc fingers (only 24 of 321, ~ 7%), which evolve quickly by shuffling their many zinc finger arrays, resulting in largely dissimilar DBD sequences (and hence, DNA-binding motifs) across organisms [ 42 ]. Weighted gene correlation network analysis (WGCNA) [ 43 ] revealed stage-specific patterns in TF expression (Fig. 3 b; Additional file 3 ). For example, Fer3, a basic Helix-Loop-Helix (bHLH) TF—previously linked to reproductive mechanisms [ 44 ]—showed increased expression in F. occidentalis adults compared to the larvae and propupae. In addition, multiple Hox genes exhibited increased expression in the propupae, which is consistent with their role in morphological development [ 45 ].
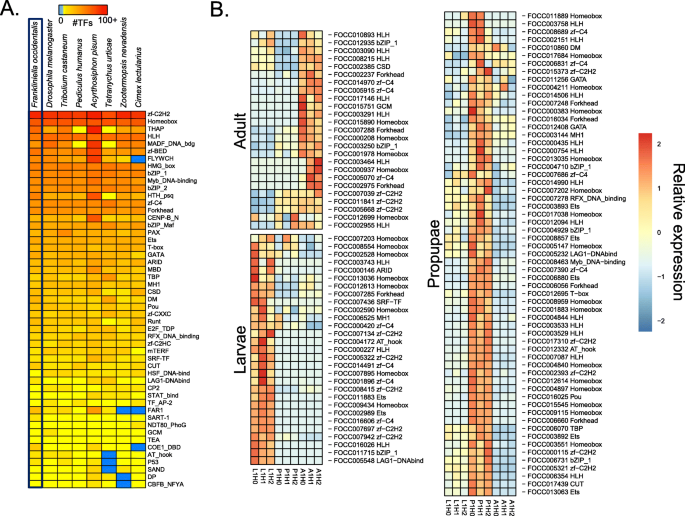
Distribution of transcription factor families across insect genomes and stage-specific expression in Frankliniella occidentalis. a Heatmap depicting the abundance of transcription factor (TF) families across a collection of insect genomes. Each entry indicates the number of TF genes for the given family in the given genome, based on presence of DNA-binding domains (DBD). Color key is depicted at the top (light blue means the TF family is completely absent)—note log (base 2) scale. Species were hierarchically clustered using average linkage clustering. F. occidentalis is boxed. See Additional file 2 : Table S5 for TF genes with predicted DBDs. b Expression of specific TFs enriched within each developmental stage (larvae, propupae, and adult) based on data presented in Additional file 3 . Sample designations: L1 = first-instar larvae, P1 = propupae, and A1 = adults (mixed males and females) of healthy cohorts (H) from three biological replicates (0, 1, 2)
Genome-wide search for putative lateral gene transfers (LGTs) of bacterial origin
Once thought to be rare, LGTs from microbes into genomes of arthropods are now considered to be relatively common [ 46 ]. Although LGTs are expected to degrade due to mutation and deletion, natural selection can lead to the evolution of functional genes from LGTs, thus expanding the genetic repertoire of the recipient species [ 47 ]. We investigated candidate LGTs in F. occidentalis using a modification of the pipeline originally developed by Wheeler et al. [ 48 ], which has been used to identify LGTs in a number of arthropod species (e.g., [ 38 , 49 , 50 ]).
Three ancient LGT events from different bacterial sources were detected in the F. occidentalis genome, involving a levanase, a mannanase, and an O-methyltransferase, with subsequent gene family expansions (Additional file 1 : Section 2, Table S2.1, Figures S2.1–S2.3) [ 24 , 28 , 38 , 48 , 51 , 52 , 53 , 54 , 55 , 56 , 57 ]. A PCR-based approach was used to confirm physical linkage between the candidate LGTs and the nearest annotated thrips genes found on the same genomic scaffolds (Additional file 1 : Section 2, Table S2.2).
Two of these LGTs show evidence of subsequent evolution into functional thrips genes, based on maintenance of an open reading frame, transcriptional activity, and a signature of purifying selection indicated by reduced levels of non-synonymous to synonymous substitution (Additional file 1 : Table S2.1). Both of these are glycoside hydrolases (GHs), which are a large class of proteins involved in carbohydrate metabolism [ 58 ]. One is a mannanase (GH5) which was acquired from a Bacillus or Paenibacillus based on phylogenetic analysis. This gene subsequently underwent expansion into three paralogs in Frankliniella . The second ancient LGT is a levanase (GH32) that has undergone duplication subsequent to transfer. The possible origin of this gene is a bacterium in the genus Streptomyces or Massilia , although the phylogenetic reconstruction precludes a clear resolution of its source. These LGTs could be important in carbohydrate metabolism and therefore impact the feeding ecology of F. occidentalis , although their actual functions remain a topic for future study.
The O-methyltransferase LGT is likely derived from a bacterium in the Silvanigrellales or a related proteobacteria in the class Oligoflexia. O-methyltransferases induce the addition of a methyl moiety to small molecules and can affect many biological processes [ 52 ]. Subsequent to transfer, the gene has expanded into a three-gene family and two show transcriptional activity based on currently available RNA sequencing data. Whether any of these copies has evolved function in F. occidentalis is less clear. There is not strong evidence for purifying selection in any of the paralogs; however, one shows a significant signature of directional selection (Additional file 1 : Section 2, Table S2.1).
All three LGT events appear to be ancient. A search of the NCBI transcriptome sequence assembly (TSA) database for Thysanoptera indicates that O-methyltransferase and levanase were acquired prior to divergence of the thrips suborders Terebrantia and Tubulifera approximately 260 MYA [ 54 ], while the mannanase was acquired after divergence of the Thripidae and Aeolothripidae approximately 175 MYA. A better understanding of LGT history in thrips will come as additional genomes and more complete phylogenies are available. Further analyses could help to elucidate their functional evolutionary roles in thrips.
Gene set annotations and analyses
Here we report on the consortium’s analysis of Frankliniella occidentalis gene sets and, in select cases, gene expression associated with four primary themes centered on interactions between phytophagous insects, plants, and their environment:
Host-locating, sensing, and neural processes;
Plant feeding and detoxification;
Innate immunity, including RNA interference; and
Development and reproduction.
Host-locating, sensing and neural processes
- Chemosensory receptors
Chemosensation is important for most insects, including thrips, and the three major gene families of chemoreceptors, the odorant and gustatory receptors (ORs and GRs) in the insect chemoreceptor superfamily [ 59 ], and the unrelated ionotropic receptors (IRs) [ 60 ], mediate most smell and taste abilities [ 61 ]. Chemosensory organs have been described on the antennae of several thrips species, and on the mouthcone, and within the precibarium and cibarium of F. occidentalis [ 5 , 8 , 62 ]. Chemosensation plays an important role in the sequence of behaviors involved in host exploration by F. occidentalis . This behavioral repertoire includes surface exploration (antennal waving, presumably perceiving olfactory cues; labial dabbing, detecting surface chemistry with paraglossal sensory pegs) (Fig. 1 c) and internal exploration (perception of plant fluids with precibarial and cibarial sensilla) (Figure 13 in [ 8 ]). The OR, GR, and IR gene families from F. occidentalis were compared with those from other representative hemipteroids, specifically the human body louse P. humanus [ 63 ], the pea aphid A. pisum [ 64 ], and the bedbug C. lectularius [ 39 ], as well as conserved representatives from D. melanogaster [ 59 , 60 ] and other insects (Additional file 1 : Section 3 [ 37 , 39 , 61 , 65 , 66 , 67 , 68 , 69 , 70 , 71 , 72 , 73 , 74 , 75 , 76 , 77 , 78 , 79 , 80 , 81 , 82 , 83 , 84 , 85 , 86 , 87 , 88 , 89 , 90 , 91 , 92 , 93 , 94 , 95 , 96 , 97 , 98 , 99 , 100 , 101 , 102 , 103 , 104 , 105 , 106 , 107 ]; Additional file 2 : Table S7; Additional file 4 ). The OR family consists of a highly conserved 1:1 ortholog, the Odorant receptor co-receptor (Orco), found in most insects, including F. occidentalis as determined here, plus a variable number of “specific” ORs that bind particular ligands. Comparable to the number reported for A. pisum [ 64 ], F. occidentalis has 84 specific OR genes and all form a divergent clade in phylogenetic analysis of the family (Additional file 1 : Figure S3.1), reflecting the generally rapid sequence divergence of ORs in insects and the divergence of thrips from other hemipteroids or Paraneoptera [ 33 ]. In addition, F. occidentalis has 102 GRs—second to the milkweed bug, Oncopeltus fasciatus (115 GR genes) [ 38 ] and third in a ranking with other well-curated hemipteran genomes [ 108 ]. Phylogenetic analysis of the F. occidentalis GRs revealed large expansions within the candidate sugar (18 genes) and carbon dioxide (30 genes) receptor subfamilies (Additional file 1 : Figure S3.2). It is unclear how the expansion of sugar receptors might be involved in Frankliniella utilization of flowers on host plants, in part because we have yet to fully understand how the eight Drosophila sugar receptors [ 59 ] are deployed to sense diverse sugars [ 94 ]. The large expansion of 30 genes in the carbon dioxide receptor subfamily is comparable to a similar expansion of this subfamily in the dampwood termite Zootermopsis nevadensis [ 109 ] and the German cockroach Blattella germanica [ 95 ], but not all are expected to be involved in perception of this gas. The F. occidentalis GR repertoire also includes a small expansion of the candidate fructose receptor subfamily to five genes compared to one in other hemipteroids. This subfamily belongs to a distinct lineage of GRs, and in D. melanogaster , which have been implicated in detecting “bitter” compounds typically from plants [ 99 ]. The remaining 49 GRs, perhaps playing a similar role in detecting “bitter” plant defensive compounds, are highly divergent from those of other hemipteroids. With indication of old and young gene duplications (Additional file 1 : Figure S3.2), this group includes a recent expansion of very similar GRs (GR54–67) perhaps involved in sensing host plant chemicals.
The IR family consists of several proteins that are conserved throughout most pterygote insects including the three known co-receptors (Ir8a, 25a, and 76b) and a set of four proteins involved in perception of temperature and humidity (Ir21a, 40a, 68a, and 93a) [ 102 ]. Like other hemipteroids and most other insects, F. occidentalis has single orthologs of each of these seven genes. This insect species also has eight members of the Ir75 clade that is commonly expanded in insects and involved in perception of acids and amines [ 103 ]. The IR family commonly has a set of divergent proteins, some encoded by intron-containing genes, while most are intronless. F. occidentalis has one intron-containing gene (Ir101) with relatives in other hemipteroids, and a large divergent clade of 167 IRs including several sets of recently duplicated genes that are encoded by mostly intronless genes (the few with single introns apparently gained them idiosyncratically after expansion of an original intronless gene) (Additional file 1 : Figure S3.3). This is a considerable expansion of IRs, with the number of IR genes in F. occidentalis being at least five times that reported for other hemipteroids (see Table 2 in [ 108 ]). By analogy with the divergent IRs of D. melanogaster that appear to function in gustation [ 106 ], these genes likely encode gustatory receptors that perhaps mediate perception of host plant chemicals and, hence, host and feeding choices.
There is considerable evidence that chemosensation is important to host, feeding, and oviposition choices made by F. occidentalis . For example, F. occidentalis detects pheromones and prefers specific plant volatiles [ 84 , 110 ]. In choice tests with diverse tomato cultivars, adult female F. occidentalis preferred fully developed flowers with sepals and petals fully open to those in earlier stages of development and opening, fed preferentially on specific portions of the flower depending on tomato cultivar, and avoided specific acylsugar exudates from Type IV trichomes of tomatoes [ 111 ]. Adult females also distinguished between acylsugar molecules, different acylsugar amounts and fatty acid profiles with differentially suppressed oviposition [ 111 , 112 , 113 ].
Vision genes
In contrast to their uniquely modified wings and mode of flight, thrips are equipped with the canonical pair of lateral compound eyes (Fig. 1 c) and three dorsal ocelli, as is typical for winged insects [ 114 ]. The success of a multitude of color and light enhanced thrips-trapping devices highlights the importance of vision for host plant recognition in this insect order [ 115 ]. For instance, female F. occidentalis have been found to exhibit preference for mature host plant flowers over senescent ones during dispersal within a radius of 4 m [ 116 ]. In phototaxis assays, F. occidentalis displayed conspicuous peak attraction to UV (355 nm) and green (525 nm) light sources in comparison to blue (405, 470 nm), yellow (590 nm), and red (660 nm) [ 117 ]. Electroretinogram studies suggested the presence of UV-, blue-, and green-sensitive photopigments in both sexes [ 117 ].
Compared to hemipteran genome species studied so far [ 38 , 39 , 66 ], the F. occidentalis genome contains a rich repertoire of the opsin G-protein-coupled receptor subfamilies that are expressed in the photoreceptors of the insect compound eye retina. This includes singleton homologs of the UV- and blue (B) opsin subfamilies as well as three homologs of the long wavelength (LW)-opsin subfamily (Additional file 2 : Table S8). The latter are closely linked within a 30-k region, indicative of a tandem gene duplication-driven gene family expansion.
Gene tree analysis provided tentative support that the F. occidentalis LW opsin cluster expansion occurred independently of the previously reported LW opsin expansions in different hemipteran groups such as water striders, shield bugs, and seed bugs (Additional file 1 : Section 4, Fig. S4.1) [ 38 , 66 , 67 , 108 , 118 , 119 , 120 , 121 , 122 , 123 , 124 , 125 , 126 , 127 , 128 , 129 , 130 ]. At the same time, the considerable protein sequence divergence of the three paralogs, which differ at over 140 amino acid sites in each pairwise comparison, indicated a more ancient origin of the cluster, potentially associated with elevated adaptive sequence change. Comparative searches for possible wavelength-sensitivity shifting/tuning substitutions paralleling those identified in the water strider LW opsin paralogs did not produce compelling evidence of candidate changes (not shown) [ 66 ]. Understanding the functional significance of the F. occidentalis LW opsin gene cluster thus requires future study.
By comparison to the differential deployment of three LW opsins in Drosophila [ 131 ], it seems likely that one F. occidentalis LW opsin paralog is specific to the ocelli, while the remaining two paralogs may be expressed in subsets of the compound eye photoreceptor cells. Overall, the presence of homologs of all three major insect retinal opsin subfamilies correlates well with the previous findings on the visual sensitivities and preferences in this species [ 117 ].
The F. occidentalis genome also contains singleton homologs of two opsin gene families generally expressed in extraretinal tissues and most often the central nervous system: c-opsin [ 123 ] and Rh7 opsin [ 122 ]. We failed to detect sequence conservation evidence for Arthropsins, the third extraretinal opsin gene family discovered in arthropods [ 121 ], despite the fact that all three extraretinal opsins are present, although at variable consistency, in hemipteran species [ 38 , 66 ].
Neuropeptide signaling
Insect genomes contain large numbers of neuropeptide and protein hormones (> 40), and their receptors, many of which play significant roles in modulating sensory signals and feeding. Neuropeptides are generally encoded by small genes and occasionally evolve rapidly including the loss and duplications of these genes in different evolutionary lineages. While a number of neuropeptides are missing in several insect genomes, the genome of F. occidentalis still seems to have a complete set of neuropeptides (Additional file 2 : Table S10), including all three allatostatin C-like peptides, which is a rather rare case in insects. Alternatively spliced exons encoding similar, but distinctive, mature peptides are also conserved: mutually exclusive exons of ion transport peptide A and B [ 132 ] and orcokinin A and B [ 133 ]. Exceptions occurred in natalisin and ACP signaling pathways [ 134 , 135 ], for which both neuropeptides and the receptors are missing in this species. A surprising finding in this genome is a second corazonin gene that encodes a slightly different version of corazonin [ 136 ]. The gene clearly arose from a duplication of the corazonin gene and it has accumulated a substantial number of changes in the sequence (Additional file 5 ). The duplicated gene encoding the corazonin precursor does not contain disruptive mutations in the open reading frame and its signal peptide is expected to be functional. The transcripts were also confirmed by RNA-seq evidence provided with the genome resources. Together, this evidence collectively suggests that it is unlikely to be a pseudogene.
Similar to the case of conserved gene number, the motif sequences of the putative mature peptides are also well conserved in F. occidentalis (Additional file 5 ). An exception in this case is found in MIP (myoinhibitory peptide or allatostatin B) [ 137 ]. While its peptide motif is highly conserved not only in insects but also in mollusks and annelids, in F. occidentalis , the C-terminal tryptophan is replaced by a phenylalanine and 23 of the 25 MIP paracopies of the precursor have this unusual sequence. The predicted presence of a disulfide bridge in the N-terminal of the longest pyrokinin is another unusual and noteworthy structural feature.
Receptors associated with the set of F. occidentalis neuropeptides and hormones were also cataloged (Additional file 2 : Table S9). In Drosophila , only a few neuropeptide genes have more than one receptor. However, in the F. occidentalis genome, there are duplicate G-protein-coupled receptors (GPCR) for SIFamide, PTH, the CRF-like diuretic hormone 44, and CNMamide. These are ancestral and are generally conserved in other insect species as single copies. What is unusual in the F. occidentalis genome is that GPCRs for trissin, vasopressin, leucokinin, and RYamide as well as the orphan GPCR moody all have local duplications, which are likely generated by recent events in this species. These recently duplicated GPCRs include receptors for neuropeptides implicated in water homeostasis: vasopressin, leucokinin, and RYamide [ 138 , 139 , 140 ], implying that osmoregulatory processes are tightly regulated in F. occidentalis .
Plant feeding
Salivary gland-associated genes.
Among piercing-sucking insects, salivation is a key component of their ability to feed on plants. Saliva may form a protective sheath for the stylets, permit intra and intercellular probing, and serve as elicitors that interact with plant defense pathways in ways that may benefit the insect (reviewed in [ 141 , 142 ]). While little is known about the function of F. occidentalis saliva, it is expected to play a key role in this insect’s capacity to feed on an extraordinarily large number of plant species and its ability to transmit viruses (reviewed in [ 20 ]). Many insect SG-associated genes, in particular those that encode proteins that elicit or suppress host defenses, are species-specific, are highly divergent, and evolve rapidly [ 143 , 144 , 145 , 146 ]. Furthermore, arthropod SG transcriptomes and proteomes have unveiled significant proportions of novel proteins, i.e., with no known homology in other, even closely related, species [ 143 , 144 ]. Among highly polyphagous arthropods (i.e., the spider mite, Tetranychus urticae , or the green peach aphid, Myzus persicae ), transcriptomic analyses revealed an especially large collection of salivary proteins and many genes that lack homology to genes of known function [ 147 , 148 , 149 , 150 ]. In light of these findings and the highly polyphagous nature of F. occidentalis , we used a comprehensive set of putative F. occidentalis salivary gland-associated genes and performed comparative analyses of RNA-seq datasets derived from salivary glands (SGs: principal salivary glands and tubular salivary glands, Fig. 1 b) [ 151 ] relative to the entire body. The analysis revealed 141 and 137 transcript sequences in SGs of F. occidentalis females and males, respectively, and 127 in a combined sex analysis that were significantly greater in abundance compared to whole-body expression. There were 123 sequences that overlapped between the three salivary gland sets (Fig. 4 a; Additional file 2 : Table S11). These 123 sequences represent 83–88% of all reads mapped in salivary gland libraries and only a maximum of 14.7% of the reads from the whole-body samples (Fig. 4 b). Many of the SG-enriched sequences (~ 69%) have fewer than one million reads mapped per salivary gland dataset and very few (11%) are highly expressed with over 2.5 million reads mapped per sequence (Fig. 4 c). Among the 123 putative SG-enriched genes, fewer than half (41%) match described proteins. The majority (~59%) are either unknown proteins (12%), i.e., matches proteins in other species that are not yet functionally characterized, or F. occidentalis -specific (46%), uncharacterized proteins with no significant match to known proteins (Additional file 2 : Table S11). Of the 14 highly expressed genes (Fig. 4 d), structural prediction analyses revealed that nine are predicted to be extracellular (among these, one has a signal peptide predicting a secreted protein), indicating that these proteins may be saliva components, and one has a predicted transmembrane domain (specific proteins denoted in Additional file 2 : Table S11, Excerpt D). At least 11 of the predicted SG-enriched proteins have provisional functions expected to be enzymatic, suggesting they likely have specific roles related to the breakdown of plant materials or response to the host during feeding (Fig. 4 e). Among these, five are predicted to be extracellularly localized, one of which has a predicted signal peptide and two are robustly predicted to be secreted proteins based on all three criteria: presence of a signal peptide cleavage site on the N terminus, predicted to be extracellularly localized, and predicted to be transmembrane proteins associated with outer membranes (details regarding function denoted in Additional file 2 : Table S11, Excerpt E). One of the proteins predicted to be secreted, the pancreatic tricylglycerol lipase-like gene (FOCC002454, original maker ID: FOCC003652-RA) and three additional thrips-specific proteins with signal peptides were included in validation of enriched expression by real-time quantitative reverse-transcription PCR. Expression analysis confirmed that the predicted SG sequences are either specifically expressed in SGs, or enriched in SGs when compared to thrips heads and bodies (Additional file 1 : Section 5, Fig. S5.1) [ 4 , 20 , 151 , 152 , 153 , 154 , 155 , 156 , 157 , 158 , 159 , 160 , 161 , 162 , 163 ]. Validation with these genes yielded a Pearson correlation coefficient of 0.845, indicating that the comparative analysis we performed accurately identified putative salivary gland-enriched sequences. The SG gene set will be very valuable in future investigations aimed at understanding the diverse diet of F. occidentalis , and the role of saliva as a vehicle for virus inoculation and possibly a means by which the insect manages plant defenses by its many hosts. Prior to the SG-enrichment analysis, other gene models encoding digestive enzymes were annotated as potential SG genes; we therefore consider these likely gut-associated genes (Additional file 2 : Table S12).
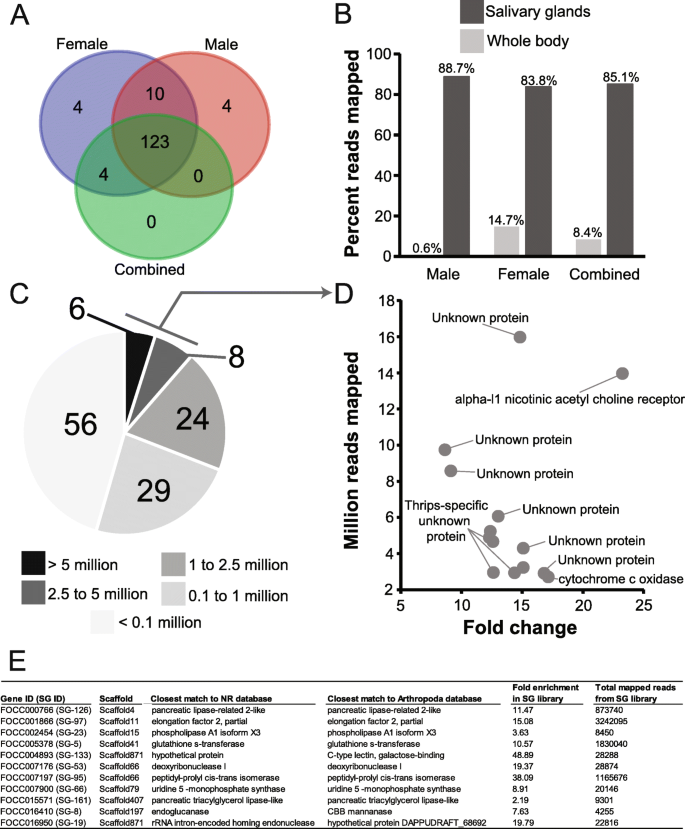
Genes/contigs with enriched expression in the salivary glands of Frankliniella occidentalis. RNA-seq reads generated from male and female principal and tubular salivary glands collectively [ 151 ] and whole bodies (this study) were used for the enrichment analysis. a Venn diagram depicting the overlap in transcript sequences enriched in the salivary glands of males, females, and combined sexes compared respectively to whole bodies. b Percent reads from salivary glands and whole-body RNA-seq datasets mapped to the putative 123 salivary gland-associated sequences. c Number of reads from the female salivary gland RNA-seq dataset mapping to each of the 123 salivary gland-associated sequences. d Reads mapped by fold change for 14 sequences with the highest number of mapped reads denoted in panel c . “Thrips-specific unknown protein” signifies hypothetical proteins with no match to proteins in other organisms and “unknown” indicates uncharacterized proteins in other arthropods. Details of expression and potential functions are denoted in Additional file 2 : Table S11 (Excerpt D). e Specific sequences with functional assignments suggesting they are enzymatic, and based on comparison with other insects systems, could be involved in plant feeding and digestion. Details of expression and potential functions are denoted in Additional file 2 : Table S11 (Excerpt E)
The thrips genome has enabled identification of SG-enriched transcripts, greatly refining our understanding of the sialotranscriptome of this highly polyphagous insect [ 151 ]. The salivary glands of thrips are of particular importance due to their role in extra-oral digestion, defense against host responses, and delivery of viruses to plants. Annotation and analysis of the SG genes revealed a suite of novel thrips genes, some encoding proteins predicted to be secreted extracellularly, thus likely components of the insect saliva, and may play roles in digestion and/or elicitation or suppression of innate plant defenses. Like other polyphagous herbivores studied to date, many of the thrips SG-enriched genes lack homology to genes of known function [ 147 , 149 ]. Further genomic and functional comparisons between polyphagous and oligophagous thrips will determine whether the high proportion of thrips-specific genes among the SG-enriched genes is related to the thrips wide host range and further enable the identification of genes that play a role in host specificity.
- Detoxification
Cytochrome P450s
Cytochrome P450s (CYPs) are a large, ancient superfamily of enzymes identified in all domains of life and are involved in the metabolism of multiple substrates with prominent roles in hormone synthesis and breakdown, development, and detoxification [ 164 , 165 ]. In agricultural systems, F. occidentalis has shown a propensity for developing resistance to insecticides commonly utilized to manage this species, and P450s have been specifically implicated in the detoxification of insecticides by F. occidentalis [ 166 , 167 ]. Within the F. occidentalis genome we identified and classified, using CYP nomenclature [ 168 ], a relatively large number of P450s—130 CYP gene models (Additional file 2 : Table S13) comprising 112 different CYP genes (Additional file 6 ). There was evidence of CYP gene clusters on some scaffolds as noted to occur in other insect genomes including D. melanogaster and T. castaneum [ 169 , 170 ]. The repertoire of F. occidentalis P450 genes represents 24 CYP families distributed across four known clans (CYP 2, 3, 4, and mito) (Additional file 1 : Section 6.1.3, Table S6.1) [ 168 , 169 , 170 , 171 , 172 , 173 , 174 , 175 , 176 , 177 , 178 ]. As with other insects, gene families in CYP clans 3 and 4 are overrepresented—these families include members frequently associated with the breakdown of toxic plant products and insecticides [ 166 ]. Family members belonging to clan 2 and mito, i.e., those associated with the synthesis and turnover of the 20-hydroxyecdysone (20E) and cuticle formation, were also represented in the genome (refer to “ Postembryonic development ” section below). The majority of annotated F. occidentalis P450s showed relatively low amino acid identity to other insect P450s, a common aspect of insect genomes [ 179 ]. In fact, of the 24 CYP families represented in the F. occidentalis genome, we identified 10 new families (= 40 of the 112 CYP genes) (Additional file 1 : Table S13), and therefore we consider these thrips-specific. Of these 40 thrips-specific CYP genes, 90% belong to clan 4, with the remaining members in clan 3, and phylogenetic analysis revealed gene duplications and subsequent expansions in gene family members of these two clans in F. occidentalis (Additional file 1 : Fig. S6.1). Given the already described importance of P450s in insecticide resistance [ 166 , 167 ], the prevalence of insecticides in the management of thrips species [ 166 ], and the multitude of plant defense compounds encountered during the their phytophagous lifestyle [ 165 ], knowledge of the diversity of P450s present within the F. occidentalis genome is likely essential for optimizing management of this important agricultural pest. The annotation of these P450 genes will enable future functional studies in F. occidentalis related to the detoxification of insecticidal and plant defense compounds.
ATP-binding cassette (ABC) transporters and carboxyl/choline esterase (CCE) genes
The ABC protein family is one of the largest protein families and present in all kingdoms of life. The majority functions as primary active transporters, hydrolyzing ATP to transport substrates across membranes. Some ABC proteins, however, are receptors or are involved in translation. The carboxyl/cholinesterase (CCE) enzyme family catalyzes the hydrolysis of carboxylesters and plays a role in many biological processes, such as neuron signaling, development, and detoxification of xenobiotics, including insecticides [ 180 , 181 , 182 ]. Forty-five and 50 putative ABC and CCE genes were annotated in the F. occidentalis genome, respectively (Additional file 2 : Table S15 and S16; Additional file 7 ). The number of F. occidentalis ABC genes is on the lower side among those reported for other insect species (Additional file 1 : Section 6.2.2, Table S6.3) [ 54 , 125 , 129 , 130 , 180 , 181 , 182 , 183 , 184 , 185 , 186 , 187 , 188 , 189 , 190 , 191 , 192 , 193 , 194 , 195 , 196 , 197 , 198 , 199 , 200 , 201 , 202 , 203 ] including Bemisia tabaci of the Hemiptera, the sister-group of the Thysanoptera [ 54 ]. Nevertheless, we did identify a lineage-specific expansion of ABCH genes within the F. occidentalis genome (Additional file 1 : Figure S6.6). Lineage-specific arthropod ABCH genes were previously shown to respond to environmental changes or xenobiotic exposure [ 183 , 187 , 190 ] and hence these ABCH genes might have a similar function in F. occidentalis . In contrast to ABC genes, the number of F. occidentalis CCE genes is among the highest of those identified in any insect species (Additional file 1 : Table S6.4). This high number of CCEs is due to a lineage-specific expansion within the dietary/detoxification class of CCEs (Additional file 1 : Figure S6.7), and with exception to Bombyx mori , it is the largest CCE expansion compared to other orders (Additional file 1 : Table S6.4). Future work should confirm whether these 28 F. occidentalis -specific CCEs are actually detoxification CCEs and whether the polyphagous nature and/or rapid development of insecticide resistance in F. occidentalis [ 200 ] might be related to this CCE expansion.
- Innate immunity
Canonical signaling pathways
Insects rely on innate immunity to respond to and limit infections by myriad microbes, viruses, and parasites encountered in their environments [ 204 , 205 , 206 , 207 , 208 , 209 , 210 , 211 ]. Here we report the annotation of genes associated with pathogen recognition, signal transduction, and execution of defense in F. occidentalis , and support these findings with a comparative analysis of immune-related transcripts in two other thrips vector species, F. fusca and Thrips palmi [ 24 , 25 , 26 ].
In total, 96 innate immune genes were curated from the genome (Additional file 2 : Table S17) [ 38 , 39 , 129 , 197 , 212 , 213 , 214 , 215 , 216 , 217 , 218 , 219 , 220 , 221 , 222 , 223 ]. Toll and JAK-STAT pathway members were well represented, and all but two members of the IMD pathway were located. Based on the number of different pathogen recognition receptors, F. occidentalis has a well-developed surveillance system—14 PGRPs and 8 GNBPs—greatly exceeding the number reported for other insects [ 38 , 224 ]. The broad plant host range and biogeography of this thysanopteran species may have expanded the repertoire of receptors capable of recognizing diverse pathogen and/or microbial-associated molecular patterns in these diverse biomes. Expansion of these surveillance systems could be due to the close contact of pupal stages with the soil environment during their development. Likewise, the melanization pathway encoded by the F. occidentalis genome is notably extensive compared to other insect genomes [ 38 , 224 ]. The melanization pathway is triggered by the binding of pathogen recognition molecules to PGRPs and is the first line of defense in insects. Prophenoloxidase (PPO) and serine proteases are the primary players of the melanization pathway. These primary players are well represented in the F. occidentalis genome, with six PPOs and serine proteases, compared to the closest plant feeding hemipteran relatives that have only two PPOs each ( Acyrthosiphon pisum and Oncopeltus fasciatus).
The most striking finding is the absence of the signal transducing molecule IMD, as well as FADD, another death domain-containing protein that acts downstream of IMD to activate transcription of antimicrobial peptides (AMPs) [ 225 ] in response to Gram-negative bacteria [ 205 ] and viruses [ 211 ]. Absence of IMD has also been reported for the hemipteran species A. pisum , Bemisia tabaci , and Diaphorina citri [ 129 , 197 , 212 , 213 , 224 ]. In Oncopeltus , IMD could not be identified by homology searches, but was identified by cloning the gene using degenerate primers [ 38 ]. IMD was also reported missing from the bedbug C. lectularius [ 39 ], but was later found using the Plautia stali IMD sequence as a query [ 214 ]. These findings in hemipterans illustrate that IMD sequences can be highly divergent and conclusions about their absence using solely a homology-based (in silico analysis) approach should be drawn with care.
It has been suggested for A. pisum that its phloem-limited diet and dependence on Gram-negative endosymbionts accounts for a generally reduced immune repertoire and the absence of IMD [ 129 , 215 , 224 ]. This does not seem valid for the polyphagous, mesophyll feeding thrips. In contrast to A. pisum , almost all other components of the IMD signaling pathway are present in Frankliniella , including two Relish molecules (Additional file 2 : Table S17). In conclusion, the apparent absence of IMD in F. occidentalis does not seem to suggest a reduced immune repertoire, but rather a different way of mediating the response to Gram-negative bacteria, possibly by Toll signaling components. In Drosophila , DAP-type peptidoglycans of Gram-negative bacteria moderately induce Toll signaling [ 216 , 217 ]. In Tenebrio molitor , PGRP-SA recognizes both Gram-positive and Gram-negative bacteria [ 218 ]. Extensive cross-reactivity of the Toll and IMD signaling pathway is the currently emerging picture from studies on other insects [ 214 , 219 , 220 ] and might have set the stage for multiple independent IMD losses in evolution [ 214 ].
Comparative analysis of innate immune transcripts in three thrips vector species
With the apparent absence of IMD and FADD genes in the F. occidentalis genome, we used a custom database of innate immune protein sequences to identify a diverse repertoire of transcripts implicating the activities of canonical humoral and cellular innate immunity from a previously assembled transcriptome of F. occidentalis adults [ 24 ] (Additional file 2 : Table S18) [ 226 , 227 , 228 , 229 , 230 , 231 , 232 , 233 , 234 ] and similarly for two other known vectors of orthotospoviruses: F. fusca [ 25 ] and Thrips palmi adults [ 26 ]. Comparative analysis revealed the occurrence of shared and species-specific innate immune-associated transcripts (Fig. 5 ; Additional file 8 ). Both IMD and FADD transcripts were apparently absent (E-value cut-off = 10 − 5 ) in all three species which agrees with the annotation of the F. occidentalis genome. Relaxing the cut-off (10 − 3 ) resulted in weak and ambiguous matches to IMD or IMD-like sequences (Additional file 1 : Section 7.4, Table S7.2) [ 38 , 212 , 224 ] of other hemipterans. Absence of transcripts encoding these two canonical genes suggests either cross-reactivity with the other immune signaling pathways or evolution of an atypical signaling pathway which is yet to be deciphered. All components of the JAK/STAT pathway were identified in all three thrips species. There appeared to be an over-representation of sequence matches to cytokine receptors in F. occidentalis and F. fusca , and while some of these may be involved in innate immunity, they likely play roles in other biological processes as well. Antioxidants, autophagy-related proteins, and inhibitors of apoptosis were well represented among the three transcriptomes. Differences in the number of immune-related transcripts identified between the species should be taken with caution—different biological and experimental factors, including thrips rearing conditions, sampling strategies, and sequencing/assembly parameters may contribute to this variation.
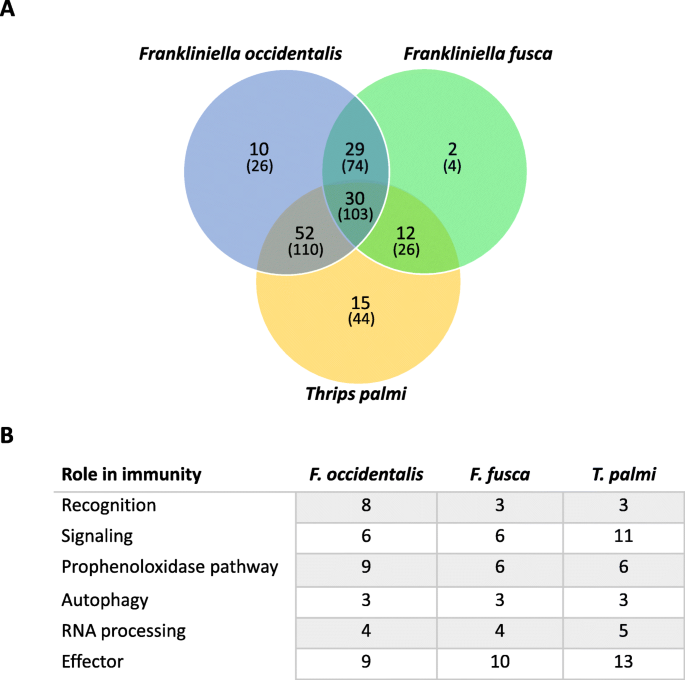
Unique and shared innate immunity-associated transcripts in three thrips vector species of orthotospoviruses. Whole-body, assembled transcriptomes obtained from published orthotospovirus-thrips RNA-seq studies [ 24 , 25 , 26 ] were mined for putative innate immune transcripts using an innate immune-associated protein database derived from ImmunoDb ( http://cegg.unige.ch/Insecta/immunodb ). a Venn diagram depicting overlap in orthologous clusters (bold) and transcripts (in parentheses) of innate immune-associated protein sequences in Frankliniella occidentalis (tomato spotted with virus), F. fusca (tomato spotted wilt virus), and Thrips palmi (capsicum chlorosis virus) using Orthovenn.v2. b Number of transcripts classified into innate immune categories (roles) and shared across all three vector species. Sequences may fall into more than one category. See Additional file 2 : Table S17 and S18, respectively, for innate immune genes and transcript sets; Additional file 8 for Orthovenn outputs
Small RNA-mediated gene silencing pathways and auxiliary genes
The RNAi-related gene set examined in this study constitutes a group of genes that are all members of a diverse range of gene (super)families that are evolutionarily unrelated but are linked based on their roles in RNAi [ 235 , 236 ]. This group includes core machinery genes for the siRNA and miRNA pathways, including several dicer and argonaute genes, drosha , pasha , aubergine , loquacious as well as several genes involved in antiviral immune response and genes encoding auxiliary proteins ( stau , maelstrom , fmr-1 , clp-1 , translin , gawky , prmt5 , hen-1 , p68 RNA helicase , ars2 , egghead ). Also, the gene encoding the transmembrane channel protein sid1 implicated in cellular uptake of dsRNA was identified in the F. occidentalis genome. F. occidentalis transmits seven described orthotospovirus species (Order Bunyavirales , Family Tospoviridae ) of economic importance, including tomato spotted wilt virus (TSWV) (reviewed in [ 22 ]). These plant-pathogenic viruses are transmitted in a persistent-propagative manner by the thrips vector, i.e., retained through molts, replicating in infected tissues, and inoculative over the lifespan of the adult. In the case of F. occidentalis , however, virus infection does not appear to have a negative effect on thrips development or fitness [ 237 , 238 ]. As RNAi is a potent innate antiviral defense in arthropods, the activities of the core cellular machinery in thrips vectors may be associated with orthotospovirus persistence.
Of the 24 RNAi-related genes queried against the genome, 23 were identified (Additional file 2 : Table S19). One gene, r2d2 , which encodes a co-factor of Dicer-2 and is therefore an element of the siRNA pathway, was not located. This could be due to the absence of r2d2 in this species, extensive divergence precluding its identification using orthologs, or location in a region of the genome that was not covered by our sequencing. Using pre-existing transcriptome sequence databases for F. occidentalis , dsRNA-binding proteins were located; however, they did not match the r2d2 sequences used as queries. For example, in a published F. occidentalis EST library of first-instar larvae [ 239 ], one sequence (GT302686) was annotated as “tar RNA binding” containing a predicted conserved domain indicative of double-stranded RNA binding (DSRM), matching a staufen-like homolog, while one sequence (contig01752) obtained from a 454 de novo-assembled transcriptome representing mixed stages of F. occidentalis matched RISC-loading complex subunit tar RNA-binding proteins [ 23 ]. In the F. occidentalis genome sequence, one gene coding for an RNA-binding protein similar to r2d2 was located, but it appeared to encode the very similar protein Loquacious (Loqs) and had a significant match (99.8%) to contig01752. Given their similarity, a phylogenetic tree was constructed with the four isoforms identified to be coded by this gene, clearly confirming that it is indeed the loqs homolog (Additional file 1 : Section 7.5, Fig. S7.8) [ 240 ].
r2d2 has been reported to be missing in other annotated winged and wingless arthropod genomes and transcriptomes. For example, r2d2 is missing from the hemipteran D. citri [ 241 ]. A recent study on the phylogenetic origin and diversification of RNAi genes reported that the gene could not be found in the transcriptomes of any of the wingless insects investigated and did not occur in some older orders of winged insects [ 240 ]. Furthermore, r2d2 also seems to be missing in non-insect arthropods. In the common shrimp Crangon crangon for example, no r2d2 could be found in the transcriptome [ 235 ] and data-mining of other Crustacea such as Daphnia pulex [ 240 ] and Artemia franciscana [ 242 ] and in the chelicerates T. urticae and Ixodes scapularis [ 147 , 240 ] also suggested that r2d2 is missing in those respective genomes. It has been suggested that in these arthropods and insects, the role of r2d2 and its interaction with Dicer-2 in the siRNA pathway may have been replaced by Loqs, which serves a similar function, interacting with Dicer-1 in the miRNA pathway. In fact, the involvement of Loqs in the siRNA pathway has been reported in the fruitfly D. melanogaster , where four dsRNA-binding proteins interacting with Dicer enzymes have been found, one encoded by the r2d2 gene and three by the loqs gene through alternative splicing. In these fruit flies, Fukunaga and Zamore [ 243 ] have shown that one of the Loqs isoforms interacts with Dicer-2 and is involved in siRNA processing. A dual role in both pathways has also been described for Loqs in Aedes aegypti [ 244 ]. Whether or not this is also the case in non-dipteran insects, such as F. occidentalis , or other arthropods is yet to be determined.
Antioxidants
Twenty-nine putative proteins in seven families related to antioxidant capacity were identified within the F. occidentalis genome (Additional file 2 : Table S20). Consequently, the suite of antioxidant proteins identified in F. occidentalis was largely as expected, and further investigation into the antioxidant system of F. occidentalis will further elucidate the players. The twenty-nine antioxidant response proteins showed high homology to related proteins in other published genomes including A. pisum , Apis mellifera , Bombyx mori , C. lectularius , D. melanogaster , P. humanus , and T. castaneum . In most comparisons, homologs in T. castaneum showed the highest degree of similarity followed by A. pisum and P. humanus .
Development
Embryonic development.
The Wnt pathway is a signal transduction pathway with fundamental regulatory roles in embryonic development in all metazoans. The emergence of several gene families of both Wnt ligands and Frizzled receptors allowed the evolution of complex combinatorial interactions with multiple layers of regulation [ 245 ]. Wnt signaling affects cell migration and segment polarity as well as segment patterning and addition in most arthropods [ 246 ]. Surveying and comparing the gene repertoire of conserved gene families within and between taxonomic groups is the first step towards understanding their function during development and evolution.
Here we curated gene models for the main components of the Wnt signaling pathway in the F. occidentalis genome (Additional file 1 : Section 8.1, Table S8.1) [ 37 , 38 , 247 , 248 , 249 , 250 , 251 , 252 ] and confirmed their orthology by phylogenetic analysis. We found 9 Wnt ligand subfamilies, three Frizzled transmembrane receptor subfamilies, the co-receptor arrow , and the downstream components armadillo/beta-catenin , dishevelled , axin , and shaggy/ GSK-3 . All of these genes, with the exception of the Frizzled family (three fz-2 paralogs), were present in single copy in the assembly. Three Wnt genes, wingless , Wnt6 , and Wnt10 , were linked on the same scaffold, reflecting the ancient arrangement of Wnt genes in Metazoa. One of the Wnt ligands, Wnt16 , has so far only been reported in the pea aphid A. pisum [ 253 ], the Russian wheat aphid Diuraphis noxia [ 254 ], and O. fasciatus [ 38 ]—adding F. occidentalis to this list suggests that the hemipteroid assemblage (clade Acercaria) has retained a Wnt ligand that was subsequently lost within the Holometabola.
Postembryonic development
Neometaboly is an atypical developmental adaptation that emerged independently in a few lineages of Paraneoptera, namely thysanopterans, Aleyrodoidea (whiteflies), and males of Coccomorpha (scales) [ 10 ]. Unlike most Hemimetabola, the transition from the penultimate juvenile stage, i.e., the second instar larva of F. occidentalis, to the adult stage involves at least one quiescent pupal stage; propupal (P1) and pupal (P2) stages in F. occidentalis (see Fig. 1 a). These pupal stages mark a period of rapid dissolution of larval structures and dramatic regeneration of the muscle tissues, nervous system, digestive tract, and eyes [ 15 ]. Underscoring the morphological transition from larvae to adults, the global network analysis in the present study revealed stage-enriched suites (modules) of co-expressed genes in F. occidentalis . There were ~ 2000–3000 stage-associated genes (transcripts) and the network assembled into 35 modules, with one, nine, and 11 modules significantly associated ( P < 0.05) with L1, P1, and adults, respectively (Fig. 6 a, Additional file 3 ). Enrichment in particular gene ontologies (provisional functions) of the stage-associated transcript sequences exemplifies the biological separation between the three stages. In L1, there was an enrichment of gene ontologies associated with metabolism and growth processes (Fig. 6 b), which was similarly reported for nymphal stages for Oncopeltus fasciatus [ 38 ]. The propupae varied widely from the larvae in that there was significant enrichment in processes associated with systems development, which included anatomical structure development, such as neuron recognition, photoreceptor cell development, and muscle structure, respiratory, and sensory system development—a reflection of the turbulent changes observed during morphogenesis of this non-feeding, quiescent stage [ 15 ] (Fig. 6 c). Adult-enriched categories implicated genes involved in transcriptional and posttranscriptional regulation of gene expression (coding and non-coding RNA-associated processes, RNA localization and RNP biogenesis), cell division (mitosis), and anatomical structure development (Fig. 6 d).
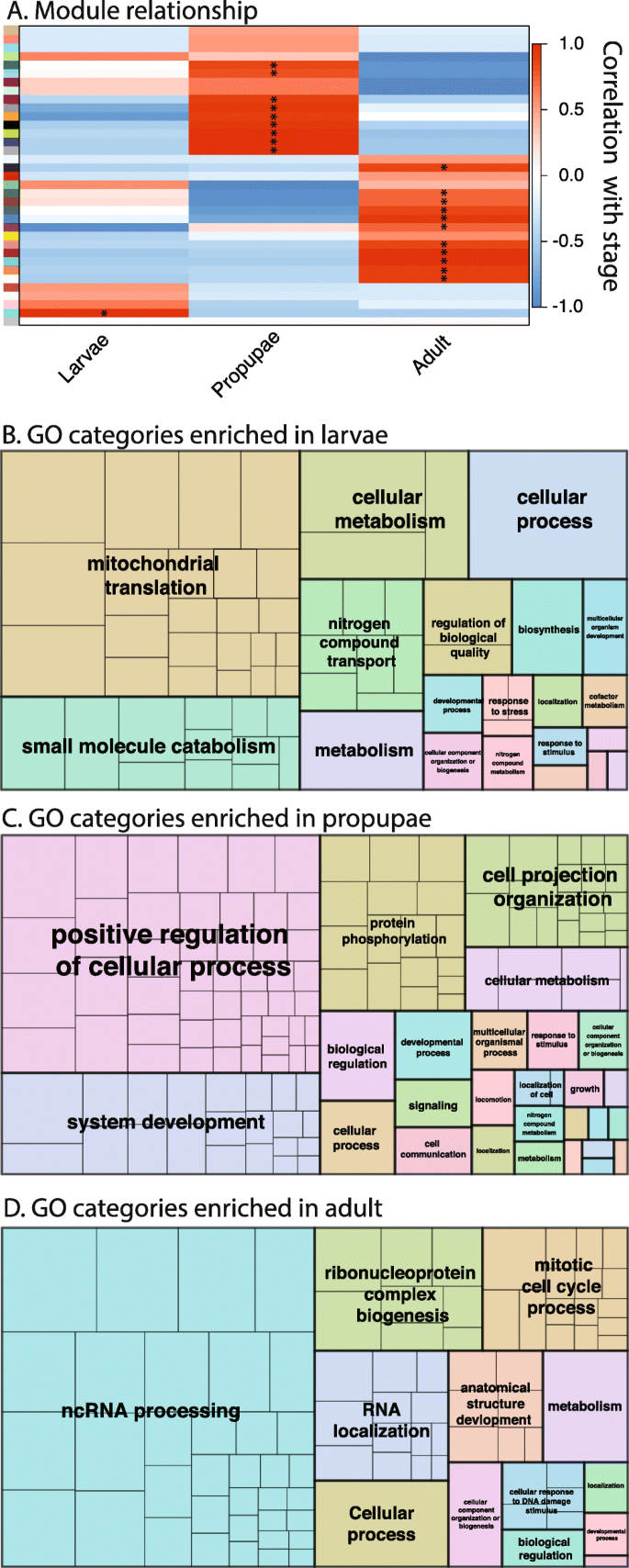
Identification of co-expressed genes (modules) and gene ontologies associated with three developmental stages of Frankliniella occidentalis . a Association between modules of co-expressed genes (colored boxes stacked on left of figure) and developmental stage, depicting the gene correlation network. Weighted gene co-expression network analysis [ 43 ] was performed on a matrix of normalized read counts (FPKM values) obtained from a published F. occidentalis RNA-seq study involving three biological replicates of healthy first-instar larvae, propupae, and adults (mixed males and females) [ 24 ]. Modules of co-expressed genes were determined by the dynamic tree cutting algorithm with a minimum of 20 genes per module. Modules that exhibited the highest correlation (red color) with a developmental stage are indicated by an asterisk (*). Transcript IDs of co-expressed genes within these significant stage-associated modules are presented in Additional file 3 . b–d REVIGO ( RE duce and V isualize G ene O ntologies, [ 255 ]) was used to visualize specific GO terms comprised of non-redundant sequences enriched in each developmental stage; sizes of delineated blocks indicate the number of genes within each GO category. Refer to Additional file 3 for more detailed REVIGO maps with identities of each GO term (block) indicated
In a more targeted approach, we curated (Additional file 2 : Table S21) and developmentally profiled expression (Additional file 2 : Table S22) of molting and metamorphosis genes. These included genes associated with the juvenile hormone (JH) and ecdysone and related signaling pathways, as well as insulin signaling and myriad transcription factors associated with the regulation of various developmental processes. Postembryonic development in insects is largely controlled by the action of two developmental hormones, JH and ecdysone. During development, JH action prevents early metamorphosis by blocking the heterochronic expression of certain ecdysone-inducible genes. JH titers maintain the juvenile-juvenile transitions, and when JH titer drops at a developmentally appropriate time, the penultimate larva/nymph develops into the pupal stage (holometabolous) or directly into the adult (hemimetabolous). Ecdysteroids (ecdysone and its derivative, 20-hydroxyecdysone (20E)) control molting at each transition. In the F. occidentalis genome, JH and ecdysone pathway genes were determined to be generally conserved. The MEKRE93 pathway [ 256 ]—consisting of the JH action transcription factors Met, Kr-h1, and E93—was fully annotated, along with the pupal-specifying gene Broad . Together, this gene battery coordinately specifies distinct developmental stages. The antimetamorphic gene Kr-h1 in F. occidentalis was previously identified [ 257 ], and the published sequence is consistent with the genome annotation. In our dataset, Met expression was associated with L1 as expected for hemi- and holometabolous insects. E93, the specifier for adult development that is thus expected to increase in expression during late nymph or propupae stages [ 256 ], was indeed upregulated and enriched in the P1 stage. In contrast, while Broad showed low expression in L1 as previously reported [ 257 ], expression was exceptionally low in P1—a finding that may be explained by P1 age at time of sampling [ 257 ]—and appeared to be associated with the young adult (Additional file 2 : Table S22). This finding differs from previous findings for F. occidentalis adults [ 257 ] and Holometabola [ 258 ]. It may be that the broad transcript quantified in our dataset was one of possibly multiple isoforms that play a role in other processes, such as nutritional or steroid signaling associated with reproduction reported for other insects [ 259 ], but this remains to be investigated. Three copies of xanthine dehydrogenase ( rosy ), a protein essential in mediating JH action in the developing abdominal epidermis of D. melanogaster [ 260 ], were identified. Of the three copies associated with F. occidentalis , xanthine dehydrogenase-2 was supported by expression data and was relatively more abundant in the adult stage. Finally, both Taiman, the steroid receptor coactivator ( AaFISC in [ 261 ], TcSRC in [ 262 ]), and FtzF1, which serves as a physical bridge between the JH receptor machinery and ecdysone, were identified with their transcripts upregulated in the P1 stage, during which these two hormones coordinately promote metamorphosis. In Aedes aegypti , [ 263 ] Ftz-F1 recruits Taiman to the ecdysteroid receptor complex to upregulate 20E-inducible genes with developmental roles [ 264 ]. Taiman knockdown in mosquitoes likewise reduces expression of the ecdysone target genes E75A and E74B and impedes ecdysone-driven morphological development [ 264 ]. E75A plays a critical role at the onset of metamorphosis [ 265 ] and requires Ftz-F1 expression; several E75A enhancers were shown to be occupied by Ftz-F1 [ 266 ]. Therefore, Ftz-F1 and Taiman expression during the F. occidentalis propupal stage is concordant with hormone-driven developmental reprogramming during transitory pupal development.
Ecdysone-associated genes were identified with varying levels of expression during development. These included 13 ecdysone cascade genes and coactivators (Additional file 2 : Table S21) and eight P450 (CYP) “Halloween” family genes, members of P450 clans 2 and mito that catalyze the biosynthesis or inactivation of 20E, were identified (Additional file 2 : Table S13; Additional file 1 : Section 6.1.3, Table S6.2) [ 176 , 177 ]. The biosynthesis pathway for 20E includes several conserved P450s [ 176 ], and as expected, these evolutionarily conserved developmental CYP genes showed some of the highest amino acid conservation observed among the collection of P450s from the F. occidentalis genome versus P450s in other insect genomes. The P450 genes responsible for the synthesis of 20E, i.e., CYP307B1/A2, CYP306A1, CYP302A, CYP315A1, and CYP314A1, were located in the F. occidentalis genome (Additional file 1 : Table S13), with four of six of these CYP transcripts differentially expressed in L1, P1, and adult stages (Additional file 2 : Table S22). CYP18A1, a key enzyme involved in the inactivation of 20E and essential for metamorphosis in D. melanogaster [ 177 ], was also identified, exhibiting high expression in the P1 stage. Cyp18A1 expression in Drosophila was during the prepupal to pupal transition [ 267 ], and in B. mori , Cyp18A1 was highly expressed in late wandering silk glands through the white prepupal stage [ 268 ]. Therefore, Cyp18A1 inactivation of ecdysone via 26-hydroxilation is a conserved phenomenon that precedes pupation across insect taxa and suggests that the propupal of F. occidentalis shares transcriptional characteristics of the white prepupal stage in these holometabolous species. In addition to these 20E-associated P450s, two copies of CYP301A1, a conserved gene shown to play a key role in the formation of adult cuticle in D. melanogaster [ 178 ], were located in the thrips genome in close proximity (on the same scaffold), possibly an indication of a tandem duplication event.
JH and ecdysone titers are tightly regulated via the action of biosynthetic and metabolic genes. Mevalonate kinase, an enzyme in the mevalonate pathway involved in JH biosynthesis in D. melanogaster and other insects, was not identified in F. occidentalis . However, CYP15A1, a single-copy P450 gene in some insects involved in the synthesis of JH, was located in the genome, and similar to A. pisum [ 269 ], there are three copies; in the F. occidentalis genome, these genes (CYP15A1/P1/P2) occur on different scaffolds (Additional file 2 : Table S13). With regard to JH degradation—which is performed by JH epoxide hydrolase (JHEH) and JH esterase (JHE) —a single obvious JHEH gene was identified in contrast to three orthologs in D. melanogaster and showed marked upregulation and enrichment in the L1 stage. The F. occidentalis genome, however, carries an additional four epoxide hydrolase orthologs, any of which may have JHEH activity—all four showed expression in L1s. Notably, several of the F. occidentalis carboxylesterase annotations meet a “diagnostic” criterion (GQS A G motif; A replaced by S in F. occidentalis ) of functional JHE proteins [ 270 ] (Additional file 1 : Section 8.2.1, Figure S8.1); however, based on the developmental expression profiles, only one of the putative JHE genes in the F. occidentalis genome is predicted as the true JHE (Additional file 2 : Table S22). Three apterous (Ap) orthologs were identified, apparently the result of tandem duplications. The apterous mutation in Drosophila results in misregulated JH production, leading to female sterility. In light of this reproductive fitness cost, expression of Ap during F. occidentalis larval and adult life—during which JH is necessary for development and reproduction—is expected. In addition to its role in promoting JH synthesis, Ap is a homeodomain protein that establishes dorsoventral boundary in the developing wing disc and Ap misexpression has a range of developmental consequences on wing morphology [ 271 ]. It is therefore intriguing to ponder a role for apterous duplications in the context of thrips’ unique wing morphology.
Many of the annotated postembryonic genes belonged to the bHLH superfamily (Additional file 1 : Section 8.2.2), transcription factors that regulate various developmental processes across all domains of life. In F. occidentalis , 45 bHLH-PAS/myc family members were conclusively annotated (Additional file 2 : Table S21). This gene superfamily showed putative duplication events—three Enhancer of split ( E(spl)-bHLH ) paralogs, two hairy orthologs, two presumed paralogs of the dimmed , and similarly, knot (syn. Collier ) (Additional file 1 : Section 8.2.3) [ 252 ]—and their expression profiles may indicate stage-specific sub/neofunctionalization (Additional file 1 : Table S22).
Cuticular proteins
Sequence motifs that are characteristic of several families of cuticle proteins [ 272 ] were used to search the genome of F. occidentalis for putative cuticle proteins. In total, 101 genes were identified, analyzed with CutProtFam-Pred, a cuticular protein family prediction tool described in Ioannidou et al. [ 273 ], and assigned to one of seven families (CPR, CPAP1, CPAP3, CPF, CPCFC, CPLCP, and TWDL) (Additional file 2 : Table S23). As with most insects, the CPR RR-1 (soft cuticle), RR-2 (hard cuticle), and unclassifiable types, constituted the largest group of cuticle protein genes in the F. occidentalis genome (Additional file 1 : Section 9, Table S9.1). The number of genes in the protein families CPR, CPAP1, CPAP3, CPCFC, and CPF were similar to the number in other insects [ 272 ]. However, the 10 genes in the TWDL family was greater than that found in most insect orders and is reminiscent of the expansion of this family observed in Diptera (Additional file 1 : Section 9, Figure S9.1). Many of the cuticle protein-encoding genes (~ 40%) were arranged in clusters of 3 to 5 genes (Additional file 1 , Table S9.2) that were primarily type-specific. However, the sizes of gene clusters were smaller than those observed in other insects, which are typically 3 to ~ 20 genes in size. Additionally, a larger portion (50–70%) of cuticle proteins is typically found in clusters in other insects—clustering of these genes could allow for the coordinated regulation of cuticle proteins and thereby facilitate the development of insecticide resistance.
Nuclear receptors
Nuclear receptors (NRs) play important roles in development, reproduction, and cell differentiation in eukaryotes. In insects, many are part of the ecdysteroid signaling cascade. Most of these NRs contain a highly conserved DNA-binding domain (DBD) and a more moderately conserved ligand-binding domain (LBD). These molecules have a very specific working mechanism, being simultaneously a transcription factor and a receptor for small amphiphilic molecules such as steroids, thyroids, vitamins, and fatty acids. In this way, they allow a direct response to certain hormone stimuli by controlling gene expression without requiring a complex cellular signaling cascade. The proteins in this superfamily are categorized into six major subfamilies (NR1-NR6) based on phylogenetic relationships, with an additional subfamily (NR0) containing non-canonical NRs usually lacking either a DBD or LBD [ 274 , 275 ]. All expected nuclear receptor genes (21 in total) commonly found in insect species were identified in the F. occidentalis genome (Additional file 2 : Table S24). All known insect members of the NR1-NR6 subfamilies were identified including the NR2E6 and NR1J1 genes that were previously reported to be missing in the hemipteran A pisum , the nearest relative to thrips and the first hemimetabolous insect to have its genome sequenced [ 129 , 276 ]. In the NR0 group, three receptors were identified (Egon, Knirps, and Knirps-like), as was the case with other members of the hemipteroid assemblage ( A. pisum and P. humanis ) and Drosophila . It is possible that the three NR0 genes found in the F. occidentalis genome are orthologous to those in Hemiptera; however, phylogenies of the arthropod NR0 genes are notoriously difficult to resolve due to the lack of semi-conserved LBD and the high divergence between these different NRs.
Reproduction
Curation and WGCNA of postembryonic developmental genes revealed members of JH, ecdysone, and insulin signaling pathways in F. occidentalis that are known to be required in other insects for vitellogenesis, functioning uniquely across taxonomic lines. For instance, ecdysone and JH have opposing functions in reproductive tissue maturation in Tribolium and Drosophila . In F. occidentalis , there were nine adult-stage, co-expressed genes implicated in oocyte development and reproductive biology (Additional file 2 : Table S22)— hydroxymethylglutaryl-CoA synthase 1 and farnesoic acid O-methyltransferase are involved in JH biosynthesis, while the others are involved in nutritional (e.g., insulin) and steroid signaling. One oddity that begs further research is the finding that methoprene tolerant (Met) was not upregulated in the sampled adult stage of F. occidentalis , since this JH receptor has roles in oocyte maturation and vitellogenesis, as well as accessory gland development and function, and in courtship behaviors. Of two lipase-3 like annotations, one was enriched in adults, while the other was enriched in larvae. Larval expression is likely related to nutritional signaling and feeding, whereas the adult transcript is likely required for reproduction.
Comparison of reproductive gene expression in male and female thrips
To identify male- and female-enriched genes, we performed a comparative RNA-seq analysis between females, males, and larvae (Additional file 9 ). Following the F. occidentalis -specific analysis, specific sets were compared to previous de novo assemblies for other thysanopteran species (Fig. 7 ). Based on these comparative analyses, 644 female-enriched, 343 male-enriched, and 181 larvae-enriched genes were identified in common among the thrips (Fig. 7 a–c). These overlapping sets for females included many factors expected to be increased in this egg generating stage, including vitellogenin and vitellogenin receptors along with other factors associated with oocyte development (Fig. 7 d, Additional file 9 : Table S1). Males had enriched expression for many factors associated with sperm generation and seminal fluid production (Fig. 7 e; Additional file 9 : Table S2). Many of these male-associated genes are hypothetical and not characterized, which is common for seminal proteins [ 277 ]. One of the male-enriched transcripts included one “myrosinase-like” transcript. Insect-expressed myrosinases have been implicated in alarm pheromone signaling in aphids [ 278 ], and the byproduct of its activity (i.e., isothiocyanates) during predation has been shown to act synergistically with the alarm pheromone E-β-farnesene [ 279 ]. By analogy to aphids, thrips-expressed myrosinases may serve roles in volatile-mediated communication and aggregation on plants [ 278 ]. The larvae datasets were enriched for aspects associated with growth and development, such as cuticle proteins (Additional file 9 : Table S3). Overall, these gene expression profiles provide putative male- and female-associated gene sets for future study .
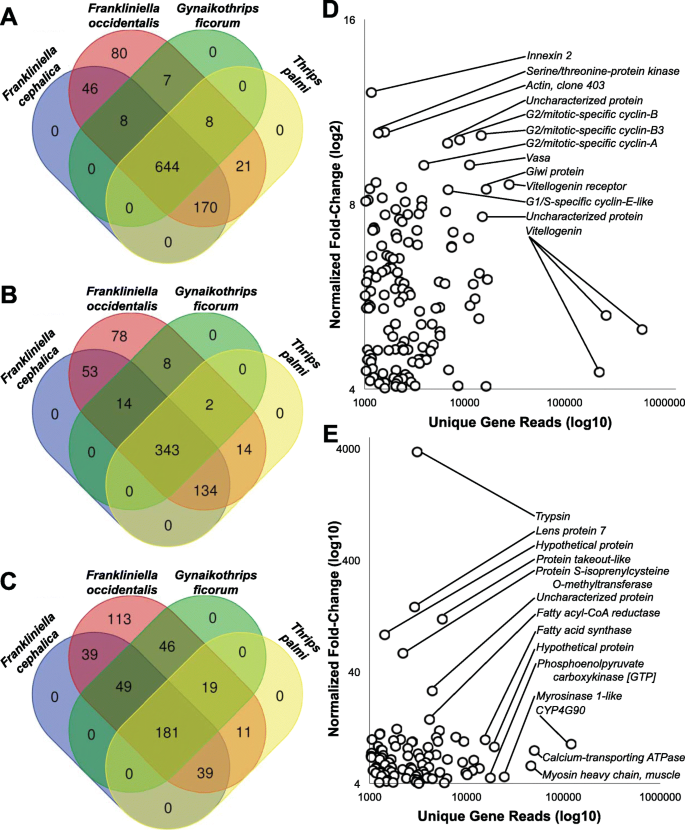
Conserved sex-specific gene expression in thrips. Genome-assembled transcripts derived from RNA-seq reads for females, males, and pre-adults (larval and pupal combined) of Frankliniella occidentalis (this study, PRJNA203209) were compared to transcripts generated de novo from publicly available RNA-seq data sets for Frankliniella cephalica (PRJNA219559), Gynaikothrips ficorum (PRJNA219563), and Thrips palmi (PRJNA219609). Venn diagrams depict the number of transcript sequences associated with a females, b males, and c pre-adult stages of thrips. Highly enriched sequences (> 1000 unique reads and > 4-fold difference) conserved in d female and e male thrips. Open circles in d and e represent highly enriched gene ontology (GO) terms; especially notable genes are labeled. See methods for enrichment criteria and Additional file 9 for sex-specific genes sets and associated normalized (TPM = transcripts per million) fold change values (relative to the other sex)
Gene family expansions
Focusing our curation efforts on selected gene sets of relevance to an herbivorous agricultural pest, we identified and characterized expansions of chemosensory receptors (ORs, GRs, IRs), vision genes (opsins), detoxification genes (CYPs, ABCHs, CCEs), innate immunity (PGRPs, GNBPs, PPOs), and cuticle-associated genes (TWDL family cuticle proteins). In order to contextualize these expansions with respect to the entire genome, we examined the outputs from the largest arthropod gene content evolution study to date [ 280 ]. Examination of Gene Ontology terms enriched among F. occidentalis gene family gains from Thomas et al. [ 280 ] revealed independent support for expansions of ORs (odorant binding, olfactory receptor activity), CYPs (oxidoreductase activity, acting on paired donors, with incorporation or reduction of molecular oxygen), ABCHs (transporter activity); PGRPs (peptidoglycan binding), GNBPs (1->3-beta-D-glucan binding), PPOs (monophenol monooxygenase activity), and TWDLs (chitin binding). PGRPs and ABCHs also appear among the 60 families with significantly rapid expansions in F. occidentalis [ 280 ]. GO terms and annotations of rapidly expanded families point to additional gains in gene families involved in lipopolysaccharide binding (putative toll receptors), inhibition of apoptosis, and chitin binding (cuticle-related). Other families with significantly rapid expansions were mostly of unknown function; however, they include several C2H2 zinc finger families, which in our analysis of transcription factors (Fig. 3 ), were determined to be the most numerous. In summation, genome-wide gains in gene families associated with chemosensation, detoxification, and innate immunity underscore the adaptive capacity of F. occidentalis to invade and thrive in diverse environments utilizing a wide array of plant hosts.
The F. occidentalis genome resources fill a missing taxon in phylogenomic-scale studies of thysanopterans and hemipterans. On the ecological level, the genome will forge new frontiers for thrips genetics and epigenetics studies, genome-wide analyses of biotic and abiotic stress encountered by this pest in diverse environments, a deeper understanding of this insect’s ability to rapidly build pesticide resistance, and identification of genes and gene products associated with plant-microbe/virus-thrips vector interactions. Importantly, the availability of this genome may also provide a means to address the challenge of determining whether F. occidentalis is a single widespread, interbreeding gene pool or a series of weakly interbreeding (even non-interbreeding) gene pools (i.e., sibling species) (Mound, personal communication, [ 29 ]). From a pest management perspective, the genome provides tools that may accelerate genome-editing for development of innovative new-generation insecticides and population suppression of targeted thrips.
The first look at gene annotations presented here points to unique features underlying the ecological success of this herbivorous pest and plant virus vector, such as the repertoire of salivary gland proteins, the majority of which are thrips-specific. Salivary components play critical roles in insect vector-plant virus interactions, including feeding, modulation of plant defenses, and virus inoculation into new hosts. Tomato spotted wilt orthotospovirus progressively invades and replicates in multiple F. occidentalis organs, including the SGs from which the virus is inoculated during feeding [ 152 ]. This intimate relationship also provides an opportunity for virus infection to modulate gene expression in insect vector SGs, which in turn may regulate insect feeding and plant defenses mediating successful inoculation. It is likely that when virus infection of SGs alters gene expression, whether genes encode proteins that facilitate feeding or mount/suppress defense, plant, insect, and/or virus may accrue substantial benefits. As we attempt to harness host plant defenses against insects and viruses and create more sustainable host plant resistance, knowledge of the F. occidentalis salivary protein repertoire provided by this genome will reveal functional roles of salivary proteins and how interplay between virus and insect modulates plant defense, insect physiology, inoculation competence, and behavior. The F. occidentalis detoxification and chemosensory genes also likely play a large role in the generalist lifestyle of this insect species. We found thrips-specific expansions within these gene families and this is consistent with the known role of these genes in perception and acceptance in diverse hosts and processing secondary metabolites. Notably, comparative transcriptomic studies of diverse plant-associated organisms revealed common themes in host-specialized transcriptomes and document the enrichment of genes that are secreted and may function as effectors, nutrient assimilation genes, and others involved in detoxification [ 148 ]. The rich and detailed information provided by this genome analysis opens broad, new avenues of basic and translational research for F. occidentalis and other thysanopteran species that will deeply impact the community of scientists and practitioners engaged in understanding this insect’s systematics, ecology, and role as a direct pest and as a vector of plant viruses.
Thrips rearing and genomic DNA isolation
A 10th generation sibling-sibling line of F. occidentalis (Pergande) was inbred for genome homozygosity from a lab colony originating from a progenitor isolated from the Kamilo Iki valley on the island of O’hau, Hawaii [ 281 ]. Thirty-one males and females were singly paired in small 1-oz clear plastic cups with lids fitted with thrips-proof-screen, and each cup contained a small cut segment of surface-disinfested green bean pod serving as the rearing and oviposition substrate. To reduce the likelihood of parthenogenetic reproduction in subsequent generations—as unfertilized female F. occidentalis produce only male progeny—early second instar larvae (L2) developing from each mating pair were removed with a fine, water-moistened paintbrush and transferred as single pairs to individual cups with a fresh cut bean to develop to adulthood. Pairs that did not develop into male-female pairs were discarded from their lineage. By the 10th generation, four inbred lines were moved to larger colony-size, 12-oz deli cups to initiate amplification of the lines, of which one thrived to establish a healthy, reproductive colony. Pools of adult females from this colony served as the biological material for genomic DNA isolation. Genomic DNA (gDNA) was isolated from eight, 10-mg subsamples of CO 2 -anesthetized females (hundreds of individuals per subsample) that were flash-frozen in liquid nitrogen, pulverized by hand with Kontes pestles (DWK Life Sciences, Thermo Fisher Scientific, Inc) then processed using the OmniPrep Genomic DNA Isolation Kit (G-Biosciences, Geno Technology, Inc., Saint Louis, MO, USA) following the manufacturer’s instructions, with the added step of 15 min room-temperature incubation of the dissolved pellet with 1 μl of LongLife RNAse (G-Biosciences) to remove residual RNA. The concentration of gDNA determined by a Nanodrop spectrophotometer (Thermo Fisher Scientific Inc.) ranged from 48 to 89 μg of DNA. Gel electrophoresis (2% agarose gel) resolved single bands of gDNA of greater than 24 kb in size. The gDNA samples were sent to the Baylor College of Medicine Human Genome Sequencing Center (BCM-HGSC) for sequencing.
Genome sequencing and assembly of Focc_1.0
Frankliniella occidentalis was one of 28 arthropod species sequenced and assembled as a part of a pilot project for the i5K arthropod genomes project at the Baylor College of Medicine Human Genome Sequencing Center [ 280 ]. As with the other i5k species, an enhanced Illumina-ALLPATHS-LG sequencing and assembly strategy for F. occidentalis enabled multiple species to be approached in parallel at reduced costs. We sequenced four libraries of nominal insert sizes 180 bp, 500 bp, 3 kb, and 8 kb. The amount of sequence generated from each of these libraries is noted in Additional file 2 : Table S1 with NCBI SRA accessions.
To prepare the 180-bp and 500-bp libraries, we used a gel-cut paired end library protocol. Briefly, 1 μg of the DNA was sheared using a Covaris S-2 system (Covaris, Inc. Woburn, MA) using the 180-bp or 500-bp program. Sheared DNA fragments were purified with Agencourt AMPure XP beads, end-repaired, dA-tailed, and ligated to Illumina universal adapters. After adapter ligation, DNA fragments were further size selected by agarose gel and PCR amplified for 6 to 8 cycles using Illumina P1 and Index primer pair and Phusion® High-Fidelity PCR Master Mix (New England Biolabs). The final library was purified using Agencourt AMPure XP beads and quality assessed by an Agilent Bioanalyzer 2100 (DNA 7500 kit) determining library quantity and fragment size distribution before sequencing.
The long mate pair libraries with 3-kb or 8-kb insert sizes were constructed according to the manufacturer’s protocol (Mate Pair Library v2 Sample Preparation Guide art # 15001464 Rev. A PILOT RELEASE). Briefly, 5 μg (for 2- and 3-kb gap size library) or 10 μg (8–10-kb gap size library) of genomic DNA was sheared to desired size fragments by Hydroshear (Digilab, Marlborough, MA), then end repaired and biotinylated. Fragment sizes between 3 and 3.7 kb (3 kb) or 8–10 kb (8 kb) were purified from 1% low melting agarose gel and then circularized by blunt-end ligation. These size selected circular DNA fragments were then sheared to 400-bp (Covaris S-2), purified using Dynabeads M-280 Streptavidin Magnetic Beads, end-repaired, dA-tailed, and ligated to Illumina PE sequencing adapters. DNA fragments with adapter molecules on both ends were amplified for 12 to 15 cycles with Illumina P1 and Index primers. Amplified DNA fragments were purified with Agencourt AMPure XP beads. Quantification and size distribution of the final library was determined before sequencing as described above.
Sequencing was performed on Illumina HiSeq2000s (Casava Version 1.8.3_V3) generating 100 bp paired end reads. Reads were assembled using ALLPATHS-LG (v35218) [ 282 ] on a large memory computer with 1 TB of RAM and further scaffolded and gap-filled using in-house tools Atlas-Link (v.1.0) [ 283 ] and Atlas gap-fill (v.2.2) [ 284 ]. The Focc_1.0 assembly was deposited in the NCBI GenBank (GCA_000697945.1) on 06/04/2014.
Automated gene annotation using a Maker 2.0 pipeline tuned for arthropods
The 28 i5K pilot genome assemblies including F. occidentalis were subjected to automatic gene annotation using a Maker 2.0 annotation pipeline tuned specifically for arthropods. The pipeline is designed to be systematic: scalable to handle 100s of genome assemblies, evidence-guided using both protein and RNA-seq evidence to guide gen models, and targeted to utilize extant information on arthropod gene sets. The core of the pipeline was a Maker 2 [ 285 ] instance, modified slightly to enable efficient running on our computational resources. The genome assembly was first subjected to de novo repeat prediction and CEGMA analysis to generate gene models for initial training of the ab initio gene predictors. Three rounds of training of the Augustus [ 286 ] and SNAP [ 287 ] gene predictors within Maker were used to bootstrap to a high-quality training set. Input protein data included 1 million peptides from a non-redundant reduction (90% identity) of Uniprot Ecdysozoa (1.25 million peptides) supplemented with proteomes from eighteen additional species ( Strigamia maritime, Tetranychus urticae, Caenorhabditis elegans, Loa loa, Trichoplax adhaerens, Amphimedon queenslandica, Strongylocentrotus purpuratus, Nematostella vectensis, Branchiostoma floridae, Ciona intestinalis, Ciona savignyi, Homo sapiens, Mus musculus, Capitella teleta, Helobdella robusta, Crassostrea gigas, Lottia gigantean, Schistosoma mansoni ) leading to a final non-redundant peptide evidence set of 1.03 million peptides. RNA-seq transcription data derived from one sample each of adult males, females, and mixed larval and pupal stages (Additional file 2 : Table S1) was used judiciously to identify exon-intron boundaries but with a heuristic script to identify and split erroneously joined gene models. We used CEGMA models for QC purposes: of 1977 CEGMA single-copy ortholog gene models, 1952 were found in the Focc_1 assembly and 1922 in the final predicted gene set—a reasonable result given the small contig sizes of the assembly. Finally, the pipeline used a nine-way homology prediction with human, Drosophila and C. elegans , and InterPro Scan5 to allocate gene names. The automated gene sets are available from the BCM-HGSC website [ 288 ] as well as the National Agriculture Library (NAL) i5k workspace sequence repository, data-share, and curation site for all i5k projects [ 67 , 289 ] where a web-browser of the genome, annotations, and supporting annotation data is accessible.
RNA evidence used to support manual genome curation
Both newly obtained and published F. occidentalis transcriptome resources were used to aid in manual annotation efforts. Using the cloud computing resources of CYVERSE (formerly iPlant Collaborative Discovery Environment) [ 290 ] and the Focc_1 genome assembly, the paired end Illumina HiSeq RNA-seq reads generated by BCM-HGSC for regular-lab-colony females, males, and pre-adults (pool of L1, L2, P1, and P2 stages) (this study, described above) were trimmed and cleaned with Prinseq-lite (version 0.20.4, [ 291 ]) and aligned and mapped to the genome with Tophat2-PE (v2.1.0). Two de novo assemblies (contigs) from published studies with F. occidentalis —one comprised of 454 sequencing reads for mixed stages of TSWV-infected and non-infected insects [ 23 ], and the other of Illumina RNA-seq reads for salivary glands of adult females and males [ 151 ] (NCBI TSA accession GAXD00000000.1), were mapped to the genome using GMAP locally [ 292 ]. These transcriptome resources were shared as RNA evidence tracks at the i5k Workspace@NAL [ 67 ]. In addition, Trinity de novo-assembled contigs [ 171 ] from Illumina HiSeq single-end reads (DNA Core Facility, University of Missouri, USA) were obtained from larval, pupal, and adult stages of F. occidentalis (+/− TSWV) (NCBI Bioproject PRJNA454326) to locate and connect fragmented cytochrome p450 (CYP) gene models using the BLAST tool at the i5k workspace.
Phylogenomic analysis of the official gene set
The OrthoDB v8 resource [ 293 ] was queried to find shared orthologs among F. occidentalis and another eight arthropods genomes; Daphnia pulex , Pediculus humanus , Acyrthosiphon pisum , Cimex lectularius , Apis mellifera , Tribolium castaneum , Danaus plexippus , and Drosophila melanogaster. Custom Perl scripts (Additional file 10 ) were used to compute the number of genes in each category shown in Fig. 2 a. For the phylogenomic analysis, only the single-copy orthologs were used to build a concatenated protein sequence alignment from which to estimate the phylogenetic tree using RAxML version 7.6.6 [ 294 ]. Briefly, a multiple sequence alignment was performed using muscle version 3.8.31 [ 295 ] for each orthologous group separately. Then, the resulting alignments were trimmed using trimAl version 1.2rev59 [ 70 ] with parameters “-w 3 -gt 0.95 -st 0.01”. The trimmed alignments were concatenated using the “seqret” program from the EMBOSS suite version 6.6.0.0 [ 296 ]. This concatenated alignment was used to build the phylogeny using RAxML version 7.6.6 with the PROTGAMMA model of amino acid substitutions and 100 bootstrap replicates.
Assessment of gene set completeness and genome assembly quality
For evaluating the completeness of the F. occidentalis official gene set, and genome assembly, we used Benchmarking Universal Single-Copy Orthologs (BUSCO) [ 297 ] of the Arthropoda gene set, which consists of 1066 single-copy genes that are present in at least 90% of selected representative arthropods (shown in Fig. 2 b). BUSCO assessments were run with the default parameters.
Community curation of the thrips genome
Seventeen groups were recruited from the i5k pilot project and thrips research community to manually curate MAKER-predicted models (Focc_v0.5.3) of gene sets of interest to thrips consortium members using the Focc_1 assembly. The consortium used Web Apollo/JBrowser tools, online training, and written guidelines made available by the National Agriculture Library (NAL) i5k workspace, along with the RNA evidence described above, to locate and correct 1694 genes, 1738 mRNAs, and 13 pseudogenes. Gene set members were located with BLAST queries of hemipteroid and Drosophila orthologs using customized parameters provided in Additional file 1 , sections entitled “Gene set manual annotations—customized strategy and phylogenetic analysis”. At the completion of the community curation period, the manually curated models were exported in gff3 format and quality-checked for formatting and curation errors, and then integrated with the MAKER-predicted gene models (Additional File 2 : Table S2) to generate a non-redundant official gene set (OGS v1.0) (Additional File 2 : Table S3). After removal of bacterial sequence contamination, the OGS v1.0 includes 16,859 genes, 16,902 mRNAs, and 13 pseudogenes.
Phylogenetic analyses of gene families
To identify cases where there is clear evidence of multiple gene duplications in F. occidentalis leading to expanded repertoires of certain gene (sub)families of special interest, we (i) invested heavily in manual curation of F. occidentalis family members; (ii) retrieved high-quality previously curated sequences from other taxa, intentionally choosing exemplar species where prior curation provides confidence that comparisons would be fair, and therefore, inferences of gene family expansions would be expected to be robust; (iii) built phylogenetic trees for each family and/or subfamily; and (iv) compared the counts and branching/clustering of genes from each species in each tree. Particular algorithms and parameters used to generate the trees are provided in Additional file 1 , sections entitled “Gene set manual annotations—customized strategy and phylogenetic analysis”, for each gene family examined phylogenetically.
Improved assembly contiguity
Well after community curation of OGSv1.0 and subsequent analyses with targeted genes sets, a second assembly was generated in an attempt to improve contig and scaffold contiguity. This assembly (Focc_2) was generated using Platanus [ 298 ] followed by haplotype collapse with Redundans [ 299 ], two tools designed for short-read genome assembly of polymorphic short-read datasets, as described by Thomas et al. [ 280 ]. While the genome assembly had better contiguity statistics, the biological utility was judged not to be as good by the community, based on attempts to transfer and identify gene models in the new assembly. Because of this, the community reverted to the original version with manually annotated gene models as described in this paper, and this version (Focc_3.0) has been designated GCA_000697945.4 in NCBI. Focc_2.0 was deposited in NCBI as GCA_000697945.3 on 12/15/17.
Identification of transcription factors
We identified likely transcription factors (TFs) by scanning the amino acid sequences of predicted protein-coding genes for putative DNA-binding domains (DBDs), and when possible, we predicted the DNA-binding specificity of each TF using the procedures described in Weirauch et al. [ 300 ]. Briefly, we scanned all protein sequences for putative DBDs using the 81 Pfam [ 301 ] models listed in Weirauch and Hughes [ 302 ] and the HMMER tool [ 303 ], with the recommended detection thresholds of Per-sequence Eval < 0.01 and Per-domain conditional Eval < 0.01. Each protein was classified into a family based on its DBDs and their order in the protein sequence (e.g., bZIPx1, AP2x2, Homeodomain+Pou). We then aligned the resulting DBD sequences within each family using clustalOmega [ 127 ] with default settings. For protein pairs with multiple DBDs, each DBD was aligned separately. From these alignments, we calculated the sequence identity of all DBD sequence pairs (i.e., the percent of AA residues that are exactly the same across all positions in the alignment). Using previously established sequence identify thresholds for each family [ 300 ], we mapped the predicted DNA-binding specificities by simple transfer. For example, the DBD of FOCC004897 (OGS ID) is 98% identical to the Drosophila melanogaster mirr protein (Additional file 2 : Table S5). Since the DNA-binding specificity of mirr has already been experimentally determined, and the cut-off for Homeodomain family of TFs is 70%, we can infer that FOCC004897 will have the same binding specificity as mirr.
Bacterial scaffold detection method
Bacterial scaffolds in F. occidentalis genome were identified using a modified nucleotide-based pipeline developed by Wheeler et al. [ 48 ] and as described previously [ 38 ]. Briefly, 1 kbp DNA fragments from each scaffold were searched for bacterial homologs against an in-house bacterial database containing 2100 bacterial species using BLASTn algorithm (Additional File 2 : Table S25). The bacterial database was masked for low complexity regions by NCBI Dustmasker [ 304 ], and similarity matches of bitscore above 50 were retained. To accurately determine the bacterial scaffolds, parameters including the number of bacterial matches per scaffold, proportion of the scaffold covered by bacterial matches, and total hit width (encompassing the distance between the leftmost and rightmost bacterial match in the scaffold) were considered. Candidate bacterial scaffolds were called based on a cut-off of ≥ 0.40 proportion bacterial hit width as this criterion with manual curation of the sequences. It should be noted, however, that represented in this set could be larger lateral gene transfers in relatively small scaffolds, as the latter cannot be readily identified without flanking eukaryotic sequences and/or further manual curation. The procedure for detecting bacterial scaffolds was performed twice with modifications—once during the early stages of the curation process (2015) using an “older” method [ 48 ] and a second time more recently as described above using this “new” method after the curation process ended and OGS v1.0 was frozen and submitted for publication.
Lateral gene transfer detection method
Candidate bacterial lateral gene transfers were identified using the method above for detecting bacterial scaffolds [ 38 , 48 , 304 ]. Analysis was limited to scaffolds more than 100 kb due to the need for flanking sequences to properly evaluate candidate LGTs. We examined each bacterial match with a bitscore > 75 that also showed a bitscore = 0 in a reference eukaryote database. Fragments flanking the 1-kbp positive hits were examined and combined for LGT analysis.
Manual curation was conducted on candidates surviving the initial filtering steps. Each candidate was searched with BLASTn to the NCBI nr/nt database. Sequences homologous to insect genes with the exception of a possible LGT in the common ancestor of closely related insects were removed. In cases where the matches to other insects were sporadic, the candidate was retained, as our experience has indicated that these can be independent LGTs into different lineages. The region was additionally searched with BLASTx to the NCBI nr/nt database. Sequences with no hits to multiple insect proteins were identified as an LGT candidate. The best bacterial match to the candidate was called using the NCBI nr and protein databases, and flanking genes within the scaffold were examined to determine whether they are eukaryotic or bacterial. We examined whether the LGT region was associated with an annotated gene within the insect genome, and if RNA sequencing data showed evidence of transcriptional activity in the LGT region. In a few cases, polymerase chain reactions were also conducted using primers that bridge the LGT candidate and flanking eukaryotic-like sequences (Additional file 1 : Section 2, Table S2.2).
PCR of LGT candidate flanking regions
The overlapping primers used to verify whether the potential LGTs were present in the thrips genome were designed using DNAMAN 7.0 (Lynnon Biosoft, Quebec, Canada). The PCR was carried out in a 20-μL reaction volume containing 4 μL 5x Phusion HF Buffer or 5x Phusion GC Buffer (depending on the difficulty in amplifying the target fragment), 0.4 μL 10 mM dNTPs, 1.0 μL 10 mM each primer, 0.6 μL DMSO, 0.2 μL Phusion DNA High-Fidelity DNA Polymerase (Thermo Scientific), 1.0 μL genome DNA template, and 11.8 μL ddH 2 O. The cycling program was set at 98 °C for 30 s, and then 34 cycles of 98 °C for 10 s, and 72 °C for ~ 2 min (30 s/kb), followed by a final extension step of 72 °C for 10 min and 4 °C hold. The PCR products were checked on a 1.0% gel after electrophoresis and then purified using a Wizard SV Gel and PCR Clean-Up System (Promega). The purified fragments were cloned into pGEMT Easy vector (Thermo Scientific) and transformed into DH5α competent cells. The positive transformants were cultured for plasmid purification using the E.Z.N.A. Plasmid Mini Kit I, (V-spin) (Omega Bio-tek) and then sent for sequencing by LGC genomics ( https://www.lgcgroup.com ). Overlapping sequences obtained from the different amplification reactions were then re-assembled and used to verify if the LGT was indeed present in the thrips genome.
Phylogenetic analysis and nucleotide sequence evolution of putative LGTs
The most promising LGT candidates were further analyzed by evaluating phylogenetic relationships and conducting branch specific synonymous and non-synonymous rate analysis with homologous references from NCBI to detect signatures of stabilizing or directional selection.
For phylogenetic analysis of protein sequences, the top proteins with the strongest bitscores (up to 50–60 proteins) to the translated region of the F. occidentalis proteins were aligned using muscle in MEGA. Protein alignments were assessed for misalignment and large in-del regions were removed. Phylogenetic trees were constructed by RAXML protein model “LG” with 1500 bootstrap replications. Outgroups were identified by identifying the bacterial species closest to the LGT in the constructed phylogeny and taking the bacteria protein and blasting to NCBI’s nr protein database restricted to the bacteria’s taxonomic group (e.g., family or order). Another phylogenetic tree including proteins within different genera from the bacteria taxonomic family was constructed.
To characterize nucleotide sequence evolution of the LGT and related sequences (e.g., synonymous and non-synonymous substitution rates), the translated nucleotide sequence were identified using the protein query from representative species throughout the protein tree and by comparing the similarity to the NCBI nucleotide database restricted to the specific species. The sequences obtained were then aligned using MUSCLE and large in-del regions were removed. A pruned version of the protein tree was created with the same representative sequences obtained for the nucleotide alignment. HYPHY’s BUSTED and BUSTEC (Branch-site Unrestricted Statistical Test of Episodic Diversification/Conservation), as described in [ 56 ], were both performed to validate the open reading frame using the nucleotide aligned sequences and the pruned protein tree by testing for positive and purifying selection respectively. The branch specific non-synonymous (dN) and synonymous (dS) were calculated using PAML CODEML free-model rate with fixed-branch lengths corresponding to the condensed protein tree and using the representative nucleotide alignment. Protein trees in Newick format used for the PAML, BUSTEC, and BUSTED analysis with NCBI accession numbers (Example XP_026279074.1: Frankliniella occidentalis ) and corresponding branch lengths are provided in Additional file 1 : Section 2.
Use of expression data and published transcriptomes to infer provisional functionality of genes involved in selected processes of interest
Identification of salivary gland (sg)-associated genes and enriched expression.
Using methods developed by Telleria et al. [ 305 ] and Ribeiro et al. [ 306 ], with modifications, a comparative analysis of transcript-level expression in SG tissues to whole bodies was performed on existing RNA-seq datasets to unambiguously identify SG-associated genes in the F. occidentalis genome and to identify genes/contigs exhibiting SG-enriched expression. Because feeding behaviors, tissue damage caused by feeding, and virus inoculation efficiency appear to be sexually dimorphic traits in F. occidentalis , we capitalized on male and female RNA-seq data sets that were generated to assist in gene prediction for this genome project (Additional file 2 : Table S1, NCBI SRA Accession = SRX897632 and SRX897634), and from salivary gland tissues (principal and tubular combined) of males and females (SRS549985, SRS549981, SRS549977, SRS549984, SRS549980, SRS54997) as previously published [ 151 ].
RNA-seq datasets were individually mapped to predicted genes from the F. occidentalis genome project using CLC Genomics Workbench 11.0 based upon settings previously described [ 305 ] with the exception that transcripts per million (TPM) was used as a proxy for gene expression. Fold changes were determined as the TPM for the salivary gland RNA-seq sets divided by the TPM for whole-body datasets. Baggerly’s test ( t -type test statistic) [ 307 ] followed by a false discovery rate (FDR) at 0.05 [ 308 ] was used to identify genes with significant enrichment in the salivary glands. Enriched genes were removed, and mapping and expression analyses were repeated to ensure low expressed genes were not missed. In addition, the RNA-seq data sets were mapped to the transcriptome previously generated from the salivary gland RNA-seq datasets [ 151 ], enriched contigs were identified as before, and a second mapping and analysis following removal of initially enriched contigs was utilized to identify low expressed salivary gland-enriched contigs. This secondary analysis was conducted to identify transcripts that were not predicted in the genome or may have not been present on an assembled scaffold.
Contigs and genes were compared to reduce overlap to a combined final SG-enriched sequence set that was generated. Briefly, blastn comparison was utilized to match sequences and only the longest sequence was retained if 100% matched was noted. After merging the non-overlapping predicted genes and contigs, the SG-enriched sequence was searched (BLASTx) against multiple NCBI non-redundant proteins databases including those for arthropods, hemipterans, viral, bacterial, plant, Drosophila , and the complete nr set with an expectation value (E-value) of at least 0.001. For transcripts with a blast hit with an E-value above 0.001, the identification was based upon the best match that included previously assigned biological function (lipase, cellulase, etc.). Following this process, transcripts with enriched expression in the salivary glands of male, females, and combined (males and females) relative to the entire body were compared to determine those that overlap between each set. Publicly available bioinformatics tools were used to make in silico predictions of structural features in the SG peptide sequences—SignalP (v.5.0) software [ 309 ] to determine the presence of a eukaryotic signal peptide cleavage site on the N terminus of the protein; TMHMM (v.2.0) software [ 310 ] to identify transmembrane domains of 18 amino acids or greater; and DeepLoc (v.1.0) [ 311 ] to predict cellular localization of the protein. SG proteins that were determined to contain a canonical signal peptide, to have an extracellular or cytoplasmic localization pattern, and/or a transmembrane domain associated with outer membranes were considered putative secretory proteins.
Real-time quantitative reverse-transcription PCR (qRT-PCR) was performed on a subset of putative SG gene transcripts to validate the comparative RNA-seq approach (Additional file 1 : Section 5). Frankliniella occidentalis females were obtained from a colony originally collected from the same Hawaiian isolate used in this study, and maintained on green bean pods [ 4 ]. Females were collected 48 h post-eclosion and salivary glands were surgically removed as previously described [ 151 ] to achieve three sample groups: salivary glands (PSG and TSG combined), head (minus SG), and body (i.e., carcass). Each sample group consisted of five females to ensure adequate quantities of total RNA, and six biological replications of dissection and total RNA was isolated for each sample group. Dissections were conducted at the same time of day within a 2-h window to minimize experimental variation (error) between sample groups.
Total RNA was extracted from thrips tissues using the Arcturus® PicoPure® RNA isolation kit (Life Technologies, USA), yields were determined by Nanodrop ND-1000 (Thermo Scientific, DE, USA), and 2 ng of total RNA templates was used to synthesis first-strand cDNA with 0.5 M of gene-specific reverse primers of the target gene and reference gene (see Additional file 1: Table S5.1) with the Verso™ cDNA synthesis Kit (Thermo Scientific, DE, USA), including the RT enhancer to remove residual DNA, in 10 μL reaction volumes for 60 min incubation at 45 °C, followed by a 10-min denaturation step at 85 °C. No-RT controls were also included in the cDNA step to ensure that possible contribution of DNA contamination to real-time PCR values (Cq) was negligible. Real-time PCR reaction mixtures (10 μl volumes) were prepared with 2 μL of cDNA, 0.2 μM of forward and reverse gene-specific primers, and SsoAdvanced™ Universal SYBR® Green Supermix (Bio-Rad Laboratories, CA), and run on a CFX96™ real-time PCR detection system (Bio-Rad Laboratories, CA) with an amplification protocol as follows: 95 °C for 30 s, 40 cycles of 95 °C for 10 s, 55 °C for 10 s, and 60 °C for 25 s, followed by a melting protocol to evaluate product quality (absence of primer-dimer and single peaks). Each plate was run with one biological replicate of each sample group (SGs, heads, bodies), four primer pairs (two putative SG genes and two reference genes) in separate wells, and three technical replicates of each PCR reaction. The two reference genes selected were F. occidentalis actin (Act, [ 154 ], and cytochrome oxidase 1 (COX [ 155 ]). Normalized expression of each of the four SG-enriched transcripts was calculated using the 2 −ΔCq method [ 156 ] and the geometric mean of Act/COX reference Cqs. Normalized expression was log transformed prior to one-way ANOVA followed by Tukey’s multiple comparison tests with GraphPad Prism software.
Comparative analysis of innate immune gene transcripts in three thrips vector species
One key finding of the F. occidentalis genome annotation was the apparent absence of an IMD gene—a core signal transducing protein in one of the evolutionarily conserved pathways involved in the production of antimicrobial peptides in insects—and the downstream FADD gene. With the availability of transcriptome sequences of three thrips vector species, we performed comparative analyses to mine for IMD pathway members and other core innate immunity-associated genes. A genome-enabled, transcriptome assembly representing TSWV-infected and non-infected adult F. occidentalis (PRJNA454326, [ 24 ]) and two de novo-assembled transcriptomes, one representing mixed stages of TSWV-infected and non-infected F. fusca (PRJNA385691, [ 25 ] and the other representing CaCV-infected and non-infected adult Thrips palmi (PRJNA498538, [ 26 ] were annotated against a custom made database of arthropod innate immunity genes downloaded from ImmunoDB [ 312 ] using the blastx algorithm in local BLAST+ v. 2.8.1 with an E-value cut-off of 10 − 5 . Blast annotations were filtered to retain annotations with highest bit score, lowest E-value, and longest alignment length.
Transcripts encoding immune-related genes in F. occidentalis , F. fusca , and T. palmi were translated in six frames using TransDecoder (Version5.5.0, [ 221 ]) with a minimum peptide length of 100 amino acids. Translated transcripts were annotated against the UniProt database downloaded on April 9, 2019, using Blastp with an E-value cut-off of 10 − 5 . Redundant translated proteins were removed by k-mer analysis to develop an initial training set for constructing Markov Model to discriminate between coding and non-coding regions. Predicted proteins homologous to proteins in the UniProt database were retained for further analysis. Orthologous innate immunity genes between these three species were identified by Orthovenn2, a web-tool used to identify orthologous and paralogous genes, with a pairwise sequence similarity cut-off of 10 − 5 and an inflation of 1.5 to define orthologous cluster structure [ 222 ]. Orthologous clusters were analyzed by UniProt search and GO Slim for functional annotation.
Gene expression patterns associated with postembryonic development
To aid in annotation of molting and metamorphosis genes, notably those with multiple copies and putative duplicates in the genome, and to globally characterize gene expression associated with progression from F. occidentalis larvae to adulthood, we performed weighted correlation network analysis (WGCNA) on normalized read counts (FPKM values) generated for healthy first-instar larvae (L1), propupae (P1), and adults (mixed males and females) across three biological replicates per developmental stage from a previously published RNA-seq study [ 24 ] (NCBI Bioproject PRJNA454326, SRA accessions: SRX4015378, SRX4061765, SRX4061766, SRX4159448, SRX4159449, SRX4159450, SRX4159451, SRX4159452). WGCNA is a clustering method that places highly correlated transcripts into colored modules based on similar patterns of expression across samples [ 43 ]. The resulting modules are used to form a correlation network to identify correlated genes in transcriptome-wide expression data sets (e.g., RNA-seq count data) and to identify the strength of associations (statistically significant correlations) between these groups of co-expressed genes (modules) and external sample traits of interest (developmental stage in the present study). Genes of zero variance were filtered out from the RNA-seq data in preparation for WGCNA. A scale-free topology threshold of 0.8 was used to identify the proper soft power of 16 for analysis. Adjacency matrix was calculated for signed network construction. Modules were determined by the dynamic tree cutting algorithm with a minimum of 20 genes per module. After relating modules to external sample traits, modules with the highest correlations were selected for further analysis using REVIGO [ 255 ] on stage-associated genes and gene ontologies (GO terms) to filter out functional redundancies between gene ontology (GO) terms (e.g., genes enriched in child terms that are counted in their associated parent terms) prior to visualization of semantically similar GO clusters for each developmental stage. Using a targeted approach, we repeated the WGCNA analysis on the curated molting and metamorphosis gene set using the same parameters, validating 60–85% clustering of these genes into modules discriminated by the global network analysis.
Identification of sex-specific genes
To generate a repertoire of sex-specific genes and to identify genes associated with F. occidentalis reproduction, we performed differential gene expression analyses on RNA-seq data for male, female, and pre-adult (larvae and pupae mixed) samples that were generated to assist in gene prediction for this genome project (Additional file 1 : Table S1, NCBI Bioproject: PRJNA203209, SRA accessions: SRX897632, SRX897634, SRX897633) using a previously described strategy [ 313 , 314 ]. Additional thrips species sequenced as a part of the 1KITE project ( Frankliniella cephalica , PRJNA219559; Gynaikothrips ficorum , PRJNA219563; Thrips palmi ; PRJNA219609) were used for identification of conserved sex- and stage-specific genes between these thrips species.
The F. occidentalis RNA-seq set was assessed with FastQC and trimmed with CLC Genomics. Reads were permitted to match up to five locations with only two mismatches and required at least 90% similarity at 70% of transcript length. Transcript levels were normalized to transcripts per million (TPM) and significant enrichment was determined with Baggerly’s test (beta-binomial distribution statistic) followed by Bonferroni correction (at 0.01) and a two-fold difference between samples. Gene identification was obtained through BLASTx searches against an NCBI non-redundant protein arthropod database with an E-value cut-off of 0.001. RNA-seq sets for the other thrips species were BLAST-searched against the finalized female, male, and pre-adult F. occidentalis sets (E-value ≤ 1.00 × 10 − 20 ). Venn diagrams [ 315 ] ( http://bioinformatics.psb.ugent.be/webtools/Venn/ ) were used to depict the number of shared genes in female, male, and pre-adult thrips. Fold change and the number of unique gene reads from the conserved female and male thrips genes were used to identify highly enriched genes (> 1000 unique reads, > 4-fold difference) in female and male thrips.
Availability of data and materials
The datasets supporting the conclusions of this article are publicly available at the NCBI, Bioproject PRJNA203209, and in the United States Department of Agriculture’s National Agricultural Library (NAL), AgData Commons repository [ 316 , 317 , 318 ]. Also, scaffolds, gene models, and genome browser are available at the NAL i5k workspace ( https://i5k.nal.usda.gov/Frankliniella_occidentalis ). Previously published transcriptome data used for particular analyses are publicly available at the NCBI, Bioprojects: PRJNA454326 [subset: SRX4015378, SRX4061765, SRX4061766, SRX4159448, SRX4159449, SRX4159450, SRX4159451, SRX4159452]; PRJNA219559; PRJNA219563; PRJNA219609; PRJNA234733 [subset: SRS549985, SRS549981, SRS549977, SRS549984, SRS549980, SRS54997]; PRJNA385691 [subset: SRS2169626, SRS2169627, SRS2169625]; and PRJNA498538 [subset: SRS3983472, SRS3983473, SRS3983474].
Change history
16 november 2020.
An amendment to this paper has been published and can be accessed via the original article.
Mound LA, Heming BS, Palmer JM. Phylogenetic relationships between the families of recent Thysanoptera (Insecta). Zool J Linn Soc. 1980;69:111–41.
Article Google Scholar
Ullman DE, Sherwood JL, German TL. Thrips as vectors of plant pathogens. In: Lewis TS, editor. Thrips as Crop Pests. Cambridge: CAB International; 1997. p. 539–65.
Ullman DE, Wescot DM, Hunter WB, Mau RFL. Internal anatomy and morphology of Frankliniella occidentalis (Pergande) ( Thysanoptera:Thripidae ) with special reference to interactions between thrips and Tomato spotted wilt virus. Int J Insect Morphol Embryol. 1989;18:289–310.
Ullman DE, Cho JJ, Mau RFL, Hunter WB, Westcot DM, Custer DM. Thrips-Tomato spotted wilt virus interactions: morphological, behavioral and cellular components influencing thrips transmission. In: Harris KF, editor. Advances in Disease Vector Research, vol. 9. New York: Springer-Verlag; 1992. p. 195–240.
Chapter Google Scholar
Hunter WB, Ullman DE. Analysis of mouthpart movements during feeding of Frankliniella occidentalis (Pergande) and Frankliniella schultzei Trybom (Thysanoptera: Thripidae). Int. J. Insect Morphol. Embryol. 1989;18(2–3):161–72.
Heming BS. Development of the mouthparts in embryos of Haplothrips verbasci (Osborn) (Insecta, Thysanoptera, Phlaeothripidae). J Morphol. 1980;164(3):235–63.
Article CAS PubMed Google Scholar
Heming BS. Structure, function, ontogeny, and evolution of feeding in thrips (Thysanoptera). In: Schaefer CW, Leschen RAB, editors. Functional Morphology of Insect Feeding. Lanham: Thomas Say Publications in Entomology, Entomological Society of America; 1993. p. 3–41.
Google Scholar
Hunter WB, Ullman DE. Precibarial and Cibarial chemosensilla in the western flower thrips, Frankliniella occidentalis (Pergande) (Thysanoptera: Thripidae). Int J Insect Morphol Embryol. 1994;23(2):69–83.
Lewis T. Thrips: their biology, ecology, and economic importance. New York: Academic Press; 1973.
Sehnal F, Svacha P, Zrzavy JZ. Evolution of insect metamorphosis. In: Gilbert LI, Tata JR, Atkinson BG, editors. Metamorphosis. Postembryonic reprogramming of gene expression in amphibian and insect cells. San Diego: Academic Press; 1996. p. 3–58.
Morse JG, Hoddle MS. Invasion biology of thrips. Annu Rev Entomol. 2006;51:67–89.
Reitz SR. Biology and ecology of the Western flower thrips (Thysanoptera: Thripidae): the making of a pest. Fla Entomol. 2009;92(1):7–13.
Gao Y, Lei Z, Reitz SR. Western flower thrips resistance to insecticides: detection, mechanisms and management strategies. Pest Manag Sci. 2012;68(8):1111–21.
Mota-Sanchez D, Wise JC: Arthropod Pesticide Resistance Database. 2020. https://www.pesticideresistance.org .
Moritz G. Structure, growth, and development. In: Lewis T, editor. Thrips as crop pests. Cambridge: CAB International; 1997. p. 15–63.
Parsons MW, Munkvold GP. Relationships of immature and adult thrips with silk-cut, fusarium ear rot and fumonisin B1 contamination of maize in California and Hawaii. Plant Pathol. 2010;59(6):1099–106.
Klose MJ, Sdoodee R, Teakle DS, Milne JR, Greber RS, Walter GH. Transmission of Three Strains of Tobacco Streak Ilarvirus by Different Thrips Species Using Virus-infected Pollen. J Phytopathol. 1996;144(6):281–4.
Dutta B, Barman AK, Srinivasan R, Avci U, Ullman DE, Langston DB, Gitaitis RD. Transmission of Pantoea ananatis and P. agglomerans , causal agents of center rot of onion ( Allium cepa ), by onion thrips ( Thrips tabaci ) through feces. Phytopathology. 2014;104(8):812–9.
He Z, Guo JF, Reitz SR, Lei ZR, Wu SY. A global invasion by the thrip, Frankliniella occidentalis : Current virus vector status and its management. Insect Sci. 2019;00:1–20.
Montero-Astúa M, Stafford-Banks C, Badillo-Vargas IE, Rotenberg D, Ullman DE, Whitfield AE. Tospovirus-thrips biology. In: Brown JK, editor. Vector-mediated transmission of plant pathogens. St Paul: APS Press; 2016. p. 289–308.
Oliver JE, Whitfield AE. The genus Tospovirus: emerging bunyaviruses that threaten food security. Annu Rev Virol. 2016;3(1):101–24.
Rotenberg D, Whitfield AE. Molecular interactions between tospoviruses and thrips vectors. Curr Opin Virol. 2018;33:191–7.
Badillo-Vargas IE, Rotenberg D, Schneweis D, Hiromasa Y, Tomich JM, Whitfield AE. Proteomic analysis of Frankliniella occidentalis and differentially expressed proteins in response to tomato spotted wilt virus infection. J Virol. 2012;86(16):8793–809.
Article CAS PubMed PubMed Central Google Scholar
Schneweis DJ, Whitfield AE, Rotenberg D. Thrips developmental stage-specific transcriptome response to tomato spotted wilt virus during the virus infection cycle in Frankliniella occidentalis , the primary vector. Virology. 2017;500:226–37.
Shrestha A, Champagne DE, Culbreath AK, Rotenberg D, Whitfield AE, Srinivasan R. Transcriptome changes associated with Tomato spotted wilt virus infection in various life stages of its thrips vector, Frankliniella fusca (Hinds). J Gen Virol. 2017;98(8):2156–70.
Gamage W, Shirani MK, Rotenberg D, Schneweis DJ, Tsai C, Dietzgen RG. Transcriptome-wide responses of adult melon thrips ( Thrips palmi ) associated with capsicum chlorosis virus infection. 2018;13(12):e0208538.
Chanbusarakum L, Ullman D. Characterization of bacterial symbionts in Frankliniella occidentalis (Pergande), Western flower thrips. J Invertebr Pathol. 2008;99(3):318–25.
Article PubMed Google Scholar
Facey PD, Meric G, Hitchings MD, Pachebat JA, Hegarty MJ, Chen X, Morgan LVA, Hoeppner JE, Whitten MMA, Kirk WDJ, Dyson PJ, Sheppard SK, Del Sol R. Draft genomes, phylogenetic reconstruction, and comparative genomics of two novel cohabiting bacterial symbionts isolated from Frankliniella occidentalis . Genome Biol Evol. 2015;7(8):2188–202.
Reitz SR, Gao Y, Kirk WDJ, Hoddle MS, Leiss KA, Funderburk JE. Invasion biology, ecology, and management of Western flower thrips. Annu Rev Entomol. 2020;65:17–37.
Petersen M, Armisén D, Gibbs RA, Hering L, Khila A, Mayer G, Richards S, Niehuis O, Misof B. Diversity and evolution of the transposable element repertoire in arthropods with particular reference to insects. BMC Evol Biol. 2019;19(1):11.
Article PubMed PubMed Central Google Scholar
Jacobson AL, Johnston JS, Rotenberg D, Whitfield AE, Booth W, Vargo EL, Kennedy GG. Genome size and ploidy of Thysanoptera. Insect Mol Biol. 2013;22(1):12–7.
Ding S, Wang S, He K, Jiang M, Li F. Large-scale analysis reveals that the genome features of simple sequence repeats are generally conserved at the family level in insects. BMC Genomics. 2017;18(1):848.
Article PubMed PubMed Central CAS Google Scholar
Misof B, Liu S, Meusemann K, Peters RS, Donath A, Mayer C, Frandsen PB, Ware J, Flouri T, Beutel RG, Niehuis O, Petersen M, Izquierdo-Carrasco F, Wappler T, Rust J, Aberer AJ, Aspock U, Aspock H, Bartel D, Blanke A, Berger S, Bohm A, Buckley TR, Calcott B, Chen J, Friedrich F, Fukui M, Fujita M, Greve C, Grobe P, Gu S, Huang Y, Jermiin LS, Kawahara AY, Krogmann L, Kubiak M, Lanfear R, Letsch H, Li Y, Li Z, Li J, Lu H, Machida R, Mashimo Y, Kapli P, McKenna DD, Meng G, Nakagaki Y, Navarrete-Heredia JL, Ott M, Ou Y, Pass G, Podsiadlowski L, Pohl H, von Reumont BM, Schutte K, Sekiya K, Shimizu S, Slipinski A, Stamatakis A, Song W, Su X, Szucsich NU, Tan M, Tan X, Tang M, Tang J, Timelthaler G, Tomizuka S, Trautwein M, Tong X, Uchifune T, Walzl MG, Wiegmann BM, Wilbrandt J, Wipfler B, Wong TK, Wu Q, Wu G, Xie Y, Yang S, Yang Q, Yeates DK, Yoshizawa K, Zhang Q, Zhang R, Zhang W, Zhang Y, Zhao J, Zhou C, Zhou L, Ziesmann T, Zou S, Li Y, Xu X, Zhang Y, Yang H, Wang J, Wang J, Kjer KM, Zhou X. Phylogenomics resolves the timing and pattern of insect evolution. Science. 2014;346(6210):763–7.
Krumlauf R. Hox genes in vertebrate development. Cell. 1994;78(2):191–201.
McNeill H, Yang CH, Brodsky M, Ungos J, Simon MA. Mirror encodes a novel PBX-class homeoprotein that functions in the definition of the dorsal-ventral border in the Drosophila eye. Genes Dev. 1997;11(8):1073–82.
Cavodeassi F, Modolell J, Gomez-Skarmeta JL. The Iroquois family of genes: from body building to neural patterning. Development. 2001;128(15):2847–55.
CAS PubMed Google Scholar
Richards S, Gibbs RA, Weinstock GM, Brown SJ, Denell R, Beeman RW. The genome of the model beetle and pest Tribolium castaneum . Nature. 2008;452(7190):955.
Panfilio KA, Vargas Jentzsch IM, Benoit JB, Erezyilmaz D, Suzuki Y, Colella S, Robertson HM, Poelchau MF, Waterhouse RM, Ioannidis P, Weirauch MT, Hughes DST, Murali SC, Werren JH, Jacobs CGC, Duncan EJ, Armisén D, Vreede BMI, Baa-Puyoulet P, Berger CS, Chang C, Chao H, Chen MM, Chen Y, Childers CP, Chipman AD, Cridge AG, Crumière AJJ, Dearden PK, Didion EM, Dinh H, Doddapaneni HV, Dolan A, Dugan S, Extavour CG, Febvay G, Friedrich M, Ginzburg N, Han Y, Heger P, Holmes CJ, Horn T, Hsiao Y, Jennings EC, Johnston JS, Jones TE, Jones JW, Khila A, Koelzer S, Kovacova V, Leask M, Lee SL, Lee C, Lovegrove MR, Lu H, Lu Y, Moore PJ, Munoz-Torres M, Muzny DM, Palli SR, Parisot N, Pick L, Porter ML, Qu J, Refki PN, Richter R, Rivera-Pomar R, Rosendale AJ, Roth S, Sachs L, Santos ME, Seibert J, Sghaier E, Shukla JN, Stancliffe RJ, Tidswell O, Traverso L. van dZ, Viala S, Worley KC, Zdobnov EM, Gibbs RA, Richards S: Molecular evolutionary trends and feeding ecology diversification in the Hemiptera, anchored by the milkweed bug genome. Genome Biol. 2019;20(1):64.
Benoit JB, Adelman ZN, Reinhardt K, Dolan A, Poelchau M, Jennings EC, Szuter EM, Hagan RW, Gujar H, Shukla JN, Zhu F, Mohan M, Nelson DR, Rosendale AJ, Derst C, Resnik V, Wernig S, Menegazzi P, Wegener C, Peschel N, Hendershot JM, Blenau W, Predel R, Johnston PR, Ioannidis P, Waterhouse RM, Nauen R, Schorn C, Ott MC, Maiwald F, Johnston JS, Gondhalekar AD, Scharf ME, Peterson BF, Raje KR, Hottel BA, Armisen D, Crumiere AJJ, Refki PN, Santos ME, Sghaier E, Viala S, Khila A, Ahn SJ, Childers C, Lee CY, Lin H, Hughes DST, Duncan EJ, Murali SC, Qu J, Dugan S, Lee SL, Chao H, Dinh H, Han Y, Doddapaneni H, Worley KC, Muzny DM, Wheeler D, Panfilio KA, Vargas Jentzsch IM, Vargo EL, Booth W, Friedrich M, Weirauch MT, Anderson MAE, Jones JW, Mittapalli O, Zhao C, Zhou JJ, Evans JD, Attardo GM, Robertson HM, Zdobnov EM, Ribeiro JMC, Gibbs RA, Werren JH, Palli SR, Schal C, Richards S. Unique features of a global human ectoparasite identified through sequencing of the bed bug genome. Nat Commun. 2016;7:10165.
McKenna DD, Scully ED, Pauchet Y, Hoover K, Kirsch R, Geib SM, Mitchell RF, Waterhouse RM, Ahn SJ, Arsala D, Benoit JB, Blackmon H, Bledsoe T, Bowsher JH, Busch A, Calla B, Chao H, Childers AK, Childers C, Clarke DJ, Cohen L, Demuth JP, Dinh H, Doddapaneni H, Dolan A, Duan JJ, Dugan S, Friedrich M, Glastad KM, Goodisman MA, Haddad S, Han Y, Hughes DS, Ioannidis P, Johnston JS, Jones JW, Kuhn LA, Lance DR, Lee CY, Lee SL, Lin H, Lynch JA, Moczek AP, Murali SC, Muzny DM, Nelson DR, Palli SR, Panfilio KA, Pers D, Poelchau MF, Quan H, Qu J, Ray AM, Rinehart JP, Robertson HM, Roehrdanz R, Rosendale AJ, Shin S, Silva C, Torson AS, Jentzsch IM, Werren JH, Worley KC, Yocum G, Zdobnov EM, Gibbs RA, Richards S. Genome of the Asian longhorned beetle ( Anoplophora glabripennis ), a globally significant invasive species, reveals key functional and evolutionary innovations at the beetle-plant interface. Genome Biol. 2016;17(1):227–8.
Wilbrandt J, Misof B, Panfilio KA, Niehuis O. Repertoire-wide gene structure analyses: a case study comparing automatically predicted and manually annotated gene models. BMC Genomics. 2019;20(1):753.
Najafabadi HS, Mnaimneh S, Schmitges FW, Garton M, Lam KN, Yang A, Albu M, Weirauch MT, Radovani E, Kim PM, Greenblatt J, Frey BJ, Hughes TR. C2H2 zinc finger proteins greatly expand the human regulatory lexicon. Nat Biotechnol. 2015;33(5):555–62.
Langfelder P, Horvath S. WGCNA: an R package for weighted correlation network analysis. BMC Bioinformatics. 2008;9(1):559.
Bitra K, Palli SR. The members of bHLH transcription factor superfamily are required for female reproduction in the red flour beetle, Tribolium castaneum . J Insect Physiol. 2010;56(10):1481–9.
Heffer A, Pick L. Conservation and variation in Hox genes: How insect models pioneered the evo-devo field. Annu Rev Entomol. 2013;58(1):161–79.
Dunning Hotopp JC, Clark ME, Oliveira DC, Foster JM, Fischer P, Munoz Torres MC, Giebel JD, Kumar N, Ishmael N, Wang S, Ingram J, Nene RV, Shepard J, Tomkins J, Richards S, Spiro DJ, Ghedin E, Slatko BE, Tettelin H, Werren JH. Widespread lateral gene transfer from intracellular bacteria to multicellular eukaryotes. Science. 2007;317(5845):1753–6.
Husnik F, McCutcheon JP. Functional horizontal gene transfer from bacteria to eukaryotes. Nat Rev Microbiol. 2018;16(2):67–79.
Wheeler D, Redding AJ, Werren JH. Characterization of an ancient lepidopteran lateral gene transfer. PLoS One. 2013;8(3):e59262.
Poynton HC, Hasenbein S, Benoit JB, Sepulveda MS, Poelchau MF, Hughes DST, Murali SC, Chen S, Glastad KM, Goodisman MAD, Werren JH, Vineis JH, Bowen JL, Friedrich M, Jones J, Robertson HM, Feyereisen R, Mechler-Hickson A, Mathers N, Lee CE, Colbourne JK, Biales A, Johnston JS, Wellborn GA, Rosendale AJ, Cridge AG, Munoz-Torres MC, Bain PA, Manny AR, Major KM, Lambert FN, Vulpe CD, Tuck P, Blalock BJ, Lin YY, Smith ME, Ochoa-Acuna H, Chen MM, Childers CP, Qu J, Dugan S, Lee SL, Chao H, Dinh H, Han Y, Doddapaneni H, Worley KC, Muzny DM, Gibbs RA, Richards S. The toxicogenome of Hyalella azteca : a model for sediment ecotoxicology and evolutionary toxicology. Environ Sci Technol. 2018;52(10):6009–22.
Tvedte ES, Walden KKO, McElroy KE, Werren JH, Forbes AA, Hood GR. Logsdon JM,Jr, Feder JL, Robertson HM: Genome of the parasitoid wasp Diachasma alloeum , an emerging model for ecological speciation and transitions to asexual reproduction. Genome Biol Evol. 2019;11(10):2767–73.
Wybouw N, Pauchet Y, Heckel DG, Van Leeuwen T. Horizontal gene transfer contributes to the evolution of arthropod herbivory. Genome Biol Evol. 2016;8(6):1785–801.
Liscombe DK, Louie GV, Noel JP. Architectures, mechanisms and molecular evolution of natural product methyltransferases. Nat Prod Rep. 2012;29(10):1238–50.
Sun BF, Xiao JH, He SM, Liu L, Murphy RW, Huang DW. Multiple ancient horizontal gene transfers and duplications in lepidopteran species. Insect Mol Biol. 2013;22(1):72–87.
Johnson KP, Dietrich CH, Friedrich F, Beutel RG, Wipfler B, Peters RS, Allen JM, Petersen M, Donath A, Walden KKO, Kozlov AM, Podsiadlowski L, Mayer C, Meusemann K, Vasilikopoulos A, Waterhouse RM, Cameron SL, Weirauch C, Swanson DR, Percy DM, Hardy NB, Terry I, Liu S, Zhou X, Misof B, Robertson HM, Yoshizawa K. Phylogenomics and the evolution of hemipteroid insects. Proc Natl Acad Sci USA. 2018;115(50):12775.
Wanker E, Schörgendorfer K, Schwab H. Expression of the Bacillus subtilis levanase gene in Escherichia coli and Saccharomyces cerevisiae . J Biotechnol. 1991;18(3):243–54.
Murrell B, Weaver S, Smith MD, Wertheim JO, Murrell S, Aylward A, Eren K, Pollner T, Martin DP, Smith DM, Scheffler K, Kosakovsky Pond SL. Gene-wide identification of episodic selection. Mol Biol Evol. 2015;32(5):1365–71.
Wang M. Purification, characterization, and production of β-mannanase from Bacillus subtilis TJ-102 and its application in gluco-mannooligosaccharides preparation. Eur Food Res Technol. 2013;237:399–408.
Article CAS Google Scholar
Lombard V, Golaconda Ramulu H, Drula E, Coutinho PM, Henrissat B. The carbohydrate-active enzymes database (CAZy) in 2013. Nucleic Acids Res. 2014;42(Database issue):490.
Robertson HM, Warr CG, Carlson JR. Molecular evolution of the insect chemoreceptor gene superfamily in Drosophila melanogaster . Proc Natl Acad Sci U S A. 2003;100(Suppl 2):14537–42.
Benton R, Vannice KS, Gomez-Diaz C, Vosshall LB. Variant ionotropic glutamate receptors as chemosensory receptors in Drosophila. Cell. 2009;136(1):149–62.
Joseph RM, Carlson JR. Drosophila chemoreceptors: a molecular interface between the chemical world and the brain. Trends Genet. 2015;31(12):683–95.
Hunter WB, Ullman DE. Anatomy and ultrastructure of the piercing-sucking mouthparts and Paraglossal sensilla of Frankliniella occidentalis ( Pergande ) ( Thysanoptera, Thripidae ). Int J Insect Morphol Embryol. 1992;21(1):17–35.
Kirkness EF, Haas BJ, Sun W, Braig HR, Perotti MA, Clark JM, Lee SH, Robertson HM, Kennedy RC, Elhaik E, Gerlach D, Kriventseva EV, Elsik CG, Graur D, Hill CA, Veenstra JA, Walenz B, Tubio JM, Ribeiro JM, Rozas J, Johnston JS, Reese JT, Popadic A, Tojo M, Raoult D, Reed DL, Tomoyasu Y, Kraus E, Mittapalli O, Margam VM, Li HM, Meyer JM, Johnson RM, Romero-Severson J, Vanzee JP, Alvarez-Ponce D, Vieira FG, Aguade M, Guirao-Rico S, Anzola JM, Yoon KS, Strycharz JP, Unger MF, Christley S, Lobo NF, Seufferheld MJ, Wang N, Dasch GA, Struchiner CJ, Madey G, Hannick LI, Bidwell S, Joardar V, Caler E, Shao R, Barker SC, Cameron S, Bruggner RV, Regier A, Johnson J, Viswanathan L, Utterback TR, Sutton GG, Lawson D, Waterhouse RM, Venter JC, Strausberg RL, Berenbaum MR, Collins FH, Zdobnov EM, Pittendrigh BR. Genome sequences of the human body louse and its primary endosymbiont provide insights into the permanent parasitic lifestyle. Proc Natl Acad Sci U S A. 2010;107(27):12168–73.
Smadja C, Shi P, Butlin RK, Robertson HM. Large gene family expansions and adaptive evolution for odorant and gustatory receptors in the pea aphid, Acyrthosiphon pisum . Mol Biol Evol. 2009;26(9):2073–86.
Mesquita RD, Vionette-Amaral RJ, Lowenberger C, Rivera-Pomar R, Monteiro FA, Minx P, Spieth J, Carvalho AB, Panzera F, Lawson D, Torres AQ, Ribeiro JM, Sorgine MH, Waterhouse RM, Montague MJ, Abad-Franch F, Alves-Bezerra M, Amaral LR, Araujo HM, Araujo RN, Aravind L, Atella GC, Azambuja P, Berni M, Bittencourt-Cunha PR, Braz GR, Calderón-Fernández G, Carareto CM, Christensen MB, Costa IR, Costa SG, Dansa M, Daumas-Filho CR, De-Paula IF, Dias FA, Dimopoulos G, Emrich SJ, Esponda-Behrens N, Fampa P, Fernandez-Medina RD, da Fonseca RN, Fontenele M, Fronick C, Fulton LA, Gandara AC, Garcia ES, Genta FA, Giraldo-Calderón GI, Gomes B, Gondim KC, Granzotto A, Guarneri AA, Guigó R, Harry M, Hughes DS, Jablonka W, Jacquin-Joly E, Juárez MP, Koerich LB, Lange AB, Latorre-Estivalis JM, Lavore A, Lawrence GG, Lazoski C, Lazzari CR, Lopes RR, Lorenzo MG, Lugon MD, Majerowicz D, Marcet PL, Mariotti M, Masuda H, Megy K, Melo AC, Missirlis F, Mota T, Noriega FG, Nouzova M, Nunes RD, Oliveira RL, Oliveira-Silveira G, Ons S, Orchard I, Pagola L, Paiva-Silva GO, Pascual A, Pavan MG, Pedrini N, Peixoto AA, Pereira MH, Pike A, Polycarpo C, Prosdocimi F, Ribeiro-Rodrigues R, Robertson HM, Salerno AP, Salmon D, Santesmasses D, Schama R, Seabra-Junior ES, Silva-Cardoso L, Silva-Neto MA, Souza-Gomes M, Sterkel M, Taracena ML, Tojo M, Tu ZJ, Tubio JM, Ursic-Bedoya R, Venancio TM, Walter-Nuno AB, Wilson D, Warren WC, Wilson RK, Huebner E, Dotson EM, Oliveira PL. Genome of Rhodnius prolixus , an insect vector of Chagas disease, reveals unique adaptations to hematophagy and parasite infection. Proc Natl Acad Sci U S A. 2015;112(48):14936–41.
Armisén D, Rajakumar R, Friedrich M, Benoit JB, Robertson HM, Panfilio KA, Ahn S, Poelchau MF, Chao H, Dinh H, Doddapaneni HV, Dugan S, Gibbs RA, Hughes DST, Han Y, Lee SL, Murali SC, Muzny DM, Qu J, Worley KC, Munoz-Torres M, Abouheif E, Bonneton F, Chen T, Chiang L, Childers CP, Cridge AG, Crumière AJJ, Decaras A, Didion EM, Duncan EJ, Elpidina EN, Favé M, Finet C, Jacobs CGC, Cheatle Jarvela AM, Jennings EC, Jones JW, Lesoway MP, Lovegrove MR, Martynov A, Oppert B, Lillico-Ouachour A, Rajakumar A, Refki PN, Rosendale AJ, Santos ME, Toubiana W, van dZ, Vargas Jentzsch IM, Lowman AV, Viala S, Richards S, Khila A. The genome of the water strider Gerris buenoi reveals expansions of gene repertoires associated with adaptations to life on the water. BMC Genomics. 2018;19(1):832.
Poelchau M, Childers C, Moore G, Tsavatapalli V, Evans J, Lee CY, Lin H, Lin JW, Hackett K. The i5k Workspace@NAL--enabling genomic data access, visualization and curation of arthropod genomes. Nucleic Acids Res. 2015;43(Database issue):714.
Larkin MA, Blackshields G, Brown NP, Chenna R, McGettigan PA, McWilliam H, Valentin F, Wallace IM, Wilm A, Lopez R, Thompson JD, Gibson TJ, Higgins DG. Clustal W and Clustal X version 2.0. Bioinformatics. 2007;23(21):2947–8.
Croset V, Rytz R, Cummins SF, Budd A, Brawand D, Kaessmann H, Gibson TJ, Benton R. Ancient protostome origin of chemosensory ionotropic glutamate receptors and the evolution of insect taste and olfaction. PLoS Genet. 2010;6(8):e1001064.
Capella-Gutiérrez S, Silla-Martínez JM, Gabaldón T. trimAl: a tool for automated alignment trimming in large-scale phylogenetic analyses. Bioinformatics. 2009;25(15):1972–3.
Guindon S, Dufayard JF, Lefort V, Anisimova M, Hordijk W, Gascuel O. New algorithms and methods to estimate maximum-likelihood phylogenies: assessing the performance of PhyML 3.0. Syst Biol. 2010;59(3):307–21.
Missbach C, Dweck HK, Vogel H, Vilcinskas A, Stensmyr MC, Hansson BS, Grosse-Wilde E. Evolution of insect olfactory receptors. Elife. 2014;3:e02115.
Brand P, Robertson HM, Lin W, Pothula R, Klingeman WE, Jurat-Fuentes JL, Johnson BR. The origin of the odorant receptor gene family in insects. Elife. 2018;7 https://doi.org/10.7554/eLife.38340 .
Robertson HM. Molecular evolution of the major arthropod chemoreceptor gene families. Annu Rev Entomol. 2019;64:227–42.
Teulon DAJ, Penman DR, Ramakers PMJ. Volatile chemicals for thrips (Thysanoptera: Thripidae) host finding and applications for thrips pest management. J Econ Entomol. 1993;86(5):1405–15.
Koschier EH, De Kogel WJ, Visser JH. Assessing the attractiveness of volatile plant compounds to Western flower thrips Frankliniella occidentalis . J Chem Ecol. 2000;26(12):2643–55.
Marullo R, Mound L, editors. Proceedings of the Reggio Calabria, Italy: 2–7 July 2001. Canberra: Australian National Insect Collection; 2002.
Mainali B, Lim UT. Behavioral response of Western flower thrips to visual and olfactory cues. J Insect Behav. 2011;24:436–46.
Cao Y, Zhi J, Cong C, Margolies DC. Olfactory cues used in host selection by Frankliniella occidentalis (Thysanoptera: Thripidae) in relation to host suitability. J Insect Behav. 2014;27(1):41–56.
Silva R, Walter GH, Wilson LJ, Furlong MJ. Effect of the postfeeding interval on olfactory responses of thrips to herbivore-induced cotton plants. Insect Sci. 2016;23(6):881–92.
Abdullah ZS, Ficken KJ, Greenfield BP, Butt TM. Innate responses to putative ancestral hosts: is the attraction of Western flower thrips to pine pollen a result of relict olfactory receptors? J Chem Ecol. 2014;40(6):534–40.
Teerling CR, Pierce HDJ, Borden JH, Gillespie DR. Identification and bioactivity of alarm pheromone in the western flower thrips, Frankliniella occidentalis . J Chem Ecol. 1993;19(4):681–97.
de Bruijn PJ, Egas M, Janssen A, Sabelis MW. Pheromone-induced priming of a defensive response in Western flower thrips. J Chem Ecol. 2006;32(7):1599–603.
Article PubMed CAS Google Scholar
Hamilton JG, Hall DR, Kirk WD. Identification of a male-produced aggregation pheromone in the western flower thrips Frankliniella occidentalis . J Chem Ecol. 2005;31(6):1369–79.
De Kogel WJ, Van Deventer P. Intraspecific attraction in the western flower thrips, Frankliniella occidentalis ; indications for a male sex pheromone. Entomol Exp Appl. 2003;107(1):87–9.
Kirk WD, Hamilton JG. Evidence for a male-produced sex pheromone in the western flower thrips Frankliniella occidentalis . J Chem Ecol. 2004;30(1):167–74.
Olaniran OA, Sudhakar AV, Drijfhout FP, Dublon IA, Hall DR, Hamilton JG, Kirk WD. A male-predominant cuticular hydrocarbon, 7-methyltricosane, is used as a contact pheromone in the western flower thrips Frankliniella occidentalis . J Chem Ecol. 2013;39(4):559–68.
Robertson HM. The insect chemoreceptor superfamily is ancient in animals. Chem Senses. 2015;40(9):609–14.
Saina M, Busengdal H, Sinigaglia C, Petrone L, Oliveri P, Rentzsch F, Benton R. A cnidarian homologue of an insect gustatory receptor functions in developmental body patterning. Nat Commun. 2015;6:6243.
Eyun SI, Soh HY, Posavi M, Munro JB, Hughes DST, Murali SC, Qu J, Dugan S, Lee SL, Chao H, Dinh H, Han Y, Doddapaneni H, Worley KC, Muzny DM, Park EO, Silva JC, Gibbs RA, Richards S, Lee CE. Evolutionary history of chemosensory-related gene families across the Arthropoda. Mol Biol Evol. 2017;34(8):1838–62.
Kent LB, Robertson HM. Evolution of the sugar receptors in insects. BMC Evol Biol. 2009;9:41.
Butterwick JA, Del Mármol J, Kim KH, Kahlson MA, Rogow JA, Walz T, Ruta V. Cryo-EM structure of the insect olfactory receptor Orco. Nature. 2018;560(7719):447–52.
Jung JW, Park KW, Ahn Y, Kwon HW. Functional characterization of sugar receptors in the western honeybee, Apis mellifera . J Asia Pac Entomol. 2015;18(1):19–26.
Fujii S, Yavuz A, Slone J, Jagge C, Song X, Amrein H. Drosophila sugar receptors in sweet taste perception, olfaction, and internal nutrient sensing. Curr Biol. 2015;25(5):621–7.
Robertson HM, Baits RL, Walden KKO, Wada-Katsumata A, Schal C. Enormous expansion of the chemosensory gene repertoire in the omnivorous German cockroach Blattella germanica . J Exp Zool B Mol Dev Evol. 2018;330(5):265–78.
Robertson HM, Kent LB. Evolution of the gene lineage encoding the carbon dioxide receptor in insects. J Insect Sci. 2009;9:19.
PubMed PubMed Central Google Scholar
Ioannidis P, Simao FA, Waterhouse RM, Manni M, Seppey M, Robertson HM, Misof B, Niehuis O, Zdobnov EM. Genomic features of the damselfly Calopteryx splendens representing a sister clade to most insect orders. Genome Biol Evol. 2017;9(2):415–30.
CAS PubMed PubMed Central Google Scholar
Miyamoto T, Slone J, Song X, Amrein H. A fructose receptor functions as a nutrient sensor in the Drosophila brain. Cell. 2012;151(5):1113–25.
Weiss LA, Dahanukar A, Kwon JY, Banerjee D, Carlson JR. The molecular and cellular basis of bitter taste in Drosophila. Neuron. 2011;69(2):258–72.
Rytz R, Croset V, Benton R. Ionotropic receptors (IRs): chemosensory ionotropic glutamate receptors in Drosophila and beyond. Insect Biochem Mol Biol. 2013;43(9):888–97.
Rimal S, Lee Y. The multidimensional ionotropic receptors of Drosophila melanogaster . Insect Mol Biol. 2018;27(1):1–7.
Knecht ZA, Silbering AF, Ni L, Klein M, Budelli G, Bell R, Abuin L, Ferrer AJ, Samuel ADT, Benton R, Garrity PA. Distinct combinations of variant ionotropic glutamate receptors mediate thermosensation and hygrosensation in Drosophila. eLife. 2016;5:e17879.
Prieto-Godino LL, Rytz R, Cruchet S, Bargeton B, Abuin L, Silbering AF, Ruta V, Dal Peraro M, Benton R. Evolution of acid-sensing olfactory circuits in Drosophilids. Neuron. 2017;93(3):661–676.e6.
Koh TW, He Z, Gorur-Shandilya S, Menuz K, Larter NK, Stewart S, Carlson JR. The Drosophila IR20a clade of ionotropic receptors are candidate taste and pheromone receptors. Neuron. 2014;83(4):850–65.
Stewart S, Koh TW, Ghosh AC, Carlson JR. Candidate ionotropic taste receptors in the Drosophila larva. Proc Natl Acad Sci U S A. 2015;112(14):4195–201.
Sánchez-Alcañiz JA, Silbering AF, Croset V, Zappia G, Sivasubramaniam AK, Abuin L, Sahai SY, Münch D, Steck K, Auer TO, Cruchet S, Neagu-Maier G, Sprecher SG, Ribeiro C, Yapici N, Benton R. An expression atlas of variant ionotropic glutamate receptors identifies a molecular basis of carbonation sensing. Nat Commun. 2018;9(1):4252.
Josek T, Walden KKO, Allan BF, Alleyne M, Robertson HM. A foreleg transcriptome for Ixodes scapularis ticks: candidates for chemoreceptors and binding proteins that might be expressed in the sensory Haller's organ. Ticks Tick Borne Dis. 2018;9(5):1317–27.
Sparks ME, Bansal R, Benoit JB, Blackburn MB, Chao H, Chen M, Cheng S, Childers C, Dinh H, Doddapaneni HV, Dugan S, Elpidina EN, Farrow DW, Friedrich M, Gibbs RA, Hall B, Han Y, Hardy RW, Holmes CJ, Hughes DST, Ioannidis P, Cheatle Jarvela AM, Johnston JS, Jones JW, Kronmiller BA, Kung F, Lee SL, Martynov AG, Masterson P, Maumus F, Munoz-Torres M, Murali SC, Murphy TD, Muzny DM, Nelson DR, Oppert B, Panfilio KA, Paula DP, Pick L, Poelchau MF, Qu J, Reding K, Rhoades JH, Rhodes A, Richards S, Richter R, Robertson HM, Rosendale AJ, Tu ZJ, Velamuri AS, Waterhouse RM, Weirauch MT, Wells JT, Werren JH, Worley KC, Zdobnov EM, Gundersen-Rindal D. Brown marmorated stink bug, Halyomorpha halys (Stål), genome: putative underpinnings of polyphagy, insecticide resistance potential and biology of a top worldwide pest. BMC Genomics. 2020;21(1):227.
Terrapon N, Li C, Robertson HM, Ji L, Meng X, Booth W, Chen Z, Childers CP, Glastad KM, Gokhale K, Gowin J, Gronenberg W, Hermansen RA, Hu H, Hunt BG, Huylmans AK, Khalil SM, Mitchell RD, Munoz-Torres MC, Mustard JA, Pan H, Reese JT, Scharf ME, Sun F, Vogel H, Xiao J, Yang W, Yang Z, Yang Z, Zhou J, Zhu J, Brent CS, Elsik CG, Goodisman MA, Liberles DA, Roe RM, Vargo EL, Vilcinskas A, Wang J, Bornberg-Bauer E, Korb J, Zhang G, Liebig J. Molecular traces of alternative social organization in a termite genome. Nat Commun. 2014;5:3636.
Abdullah ZS, Butt TM. Preferences of the peripheral olfactory system of Western Flower Thrips, Frankliniella occidentalis towards stereoisomers of common plant volatiles. Chemoecology. 2015;25(1):47–51.
Ben-Mahmoud S, Smeda JR, Chappell TM, Stafford-Banks C, Kaplinsky CH, Anderson T, Mutschler MA, Kennedy GG, Ullman DE. Acylsugar amount and fatty acid profile differentially suppress oviposition by western flower thrips, Frankliniella occidentalis , on tomato and interspecific hybrid flowers. PLoS One. 2018;13(7):e0201583.
Ben-Mahmoud S, Anderson T, Chappell TM, Smeda JR, Mutschler MA, Kennedy GG, De Jong DM, Ullman DE. A thrips vector of tomato spotted wilt virus responds to tomato acylsugar chemical diversity with reduced oviposition and virus inoculation. Sci Rep. 2019;9(1):17157.
Leckie BM, D'Ambrosio DA, Chappell TM, Halitschke R, De Jong DM, Kessler A, Kennedy GG, Mutschler MA. Differential and synergistic functionality of acylsugars in suppressing oviposition by insect herbivores. PLoS One. 2016;11(4):e0153345.
Moritz G. Thripse: Fransenflügler, Thysanoptera. Hohenwarsleben: Westarp Wissenschaften; 2006.
Matteson N, Terry I, Ascoli-Christensen A, Gilbert C. Spectral efficiency of the western flower thrips, Frankliniella occidentalis . J Insect Physiol. 1992;38(6):453–9.
Rhainds M, Shipp L. Dispersal of adult Western flower thrips (Thysanoptera: Thripidae) on chrysanthemum plants: Impact of feeding-induced senescence of inflorescences. Environ Entomol. 2003;32(5):1056–65.
Otani Y, Wakakuwa M, Arikawa K. Relationship between action spectrum and spectral sensitivity of compound eyes relating phototactic behavior of the Western flower thrips, Frankliniella occidentalis . Jpn J Appl Entomol Z. 2014;58:177–85.
Cronin TW, Porter ML. The evolution of invertebrate photopigments and photoreceptors. In: Hunt DM, Hankins MW, Collin SP, Marshall NJ, editors. Evolution of Visual and Non-visual Pigments. Boston: Springer US; 2014. p. 105–35.
Feuda R, Marlétaz F, Bentley MA, Holland PW. Conservation, duplication, and divergence of five opsin genes in insect evolution. Genome Biol Evol. 2016;8(3):579–87.
Hering L, Henze MJ, Kohler M, Kelber A, Bleidorn C, Leschke M, Nickel B, Meyer M, Kircher M, Sunnucks P, Mayer G. Opsins in onychophora (velvet worms) suggest a single origin and subsequent diversification of visual pigments in arthropods. Mol Biol Evol. 2012;29(11):3451–8.
Eriksson BJ, Fredman D, Steiner G, Schmid A. Characterisation and localisation of the opsin protein repertoire in the brain and retinas of a spider and an onychophoran. BMC Evol Biol. 2013;13(1):186.
Ni JD, Baik LS, Holmes TC, Montell C. A rhodopsin in the brain functions in circadian photoentrainment in Drosophila. Nature. 2017;545(7654):340–4.
Velarde RA, Sauer CD, Walden KK, Fahrbach SE, Robertson HM. Pteropsin: a vertebrate-like non-visual opsin expressed in the honey bee brain. Insect Biochem Mol Biol. 2005;35(12):1367–77.
Porter ML. Beyond the eye: Molecular evolution of extraocular photoreception. Integr Comp Biol. 2016;56(5):842–52.
Altschul SF, Gish W, Miller W, Myers EW, Lipman DJ. Basic local alignment search tool. J Mol Biol. 1990;215(3):403–10.
Wall DP, Fraser HB, Hirsh AE. Detecting putative orthologs. Bioinformatics. 2003;19(13):1710–1.
Sievers F, Wilm A, Dineen D, Gibson TJ, Karplus K, Li W, Lopez R, McWilliam H, Remmert M, Söding J, Thompson JD, Higgins DG. Fast, scalable generation of high-quality protein multiple sequence alignments using Clustal Omega. Mol Syst Biol. 2011;7:539.
Castresana J. Selection of conserved blocks from multiple alignments for their use in phylogenetic analysis. Mol Biol Evol. 2000;17(4):540–52.
The International Aphid Genomics Consortium. Genome Sequence of the Pea Aphid Acyrthosiphon pisum . PLoS Biol. 2010;8(2):e1000313.
Article PubMed Central CAS Google Scholar
Stamatakis A. RAxML version 8: a tool for phylogenetic analysis and post-analysis of large phylogenies. Bioinformatics. 2014;30(9):1312–3.
Rister J, Razzaq A, Boodram P, Desai N, Tsanis C, Chen H, Jukam D, Desplan C. Single-base pair differences in a shared motif determine differential Rhodopsin expression. Science (New York, N.Y.). 2015;350(6265):1258–61.
Begum K, Li B, Beeman RW, Park Y. Functions of ion transport peptide and ion transport peptide-like in the red flour beetle Tribolium castaneum . Insect Biochem Mol Biol. 2009;39(10):717–25.
Jiang H, Kim HG, Park Y. Alternatively spliced orcokinin isoforms and their functions in Tribolium castaneum . Insect Biochem Mol Biol. 2015;65:1–9.
Hauser F, Grimmelikhuijzen CJ. Evolution of the AKH/corazonin/ACP/GnRH receptor superfamily and their ligands in the Protostomia. Gen Comp Endocrinol. 2014;209:35–49.
Jiang H, Lkhagva A, Daubnerova I, Chae HS, Simo L, Jung SH, Yoon YK, Lee NR, Seong JY, Zitnan D, Park Y, Kim YJ. Natalisin, a tachykinin-like signaling system, regulates sexual activity and fecundity in insects. Proc Natl Acad Sci U S A. 2013;110(37):3526.
Veenstra JA. Isolation and structure of corazonin, a cardioactive peptide from the American cockroach. FEBS Lett. 1989;250(2):231–4.
Isaac RE, Kim YJ, Audsley N. The degradome and the evolution of Drosophila sex peptide as a ligand for the MIP receptor. Peptides. 2014;53:258–64.
Aikins MJ, Schooley DA, Begum K, Detheux M, Beeman RW, Park Y. Vasopressin-like peptide and its receptor function in an indirect diuretic signaling pathway in the red flour beetle. Insect Biochem Mol Biol. 2008;38(7):740–8.
Ohno H, Yoshida M, Sato T, Kato J, Miyazato M, Kojima M, Ida T, Iino Y. Luqin-like RYamide peptides regulate food-evoked responses in C. elegans . eLife. 2017;6:e28877.
Pannabecker T, Beyenbach K. Leucokinin-8 increases transepithelial chloride conductance in mosquito Malpighian tubules. FASEB J. 1991;5:A1107.
Stafford CA, Walker GP, Ullman DE. Hitching a ride: vector feeding and virus transmission. Commun Integr Biol. 2012;5(1):43–9.
Hogenhout SA, Bos JI. Effector proteins that modulate plant–insect interactions. Curr Opin Plant Biol. 2011;14(4):422–8.
Arcà B, Ribeiro JM. Saliva of hematophagous insects: a multifaceted toolkit. Curr Opin Insect Sci. 2018;29:102–9.
Carolan JC, Caragea D, Reardon KT, Mutti NS, Dittmer N, Pappan K, Cui F, Castaneto M, Poulain J, Dossat C, Tagu D, Reese JC, Reeck GR, Wilkinson TL, Edwards OR. Predicted effector molecules in the salivary secretome of the pea aphid ( Acyrthosiphon pisum ): a dual transcriptomic/proteomic approach. J Proteome Res. 2011;10(4):1505–18.
Boulain H, Legeai F, Guy E, Morlière S, Douglas NE, Oh J, Murugan M, Smith M, Jaquiéry J, Peccoud J, White FF, Carolan JC, Simon J, Sugio A. Fast evolution and lineage-specific gene family expansions of aphid salivary effectors driven by interactions with host-plants. Genome Biol Evol. 2018;10(6):1554–72.
Thorpe P, Cock PJA, Bos J. Comparative transcriptomics and proteomics of three different aphid species identifies core and diverse effector sets. BMC Genomics. 2016;17(1):172.
Grbić M, Van Leeuwen T, Clark RM, Rombauts S, Rouzé P, Grbić V, Osborne EJ, Dermauw W, Thi Ngoc PC, Ortego F, Hernández-Crespo P, Diaz I, Martinez M, Navajas M, Sucena É, Magalhães S, Nagy L, Pace RM, Djuranović S, Smagghe G, Iga M, Christiaens O, Veenstra JA, Ewer J, Villalobos RM, Hutter JL, Hudson SD, Velez M, Yi SV, Zeng J, Pires-daSilva A, Roch F, Cazaux M, Navarro M, Zhurov V, Acevedo G, Bjelica A, Fawcett JA, Bonnet E, Martens C, Baele G, Wissler L, Sanchez-Rodriguez A, Tirry L, Blais C, Demeestere K, Henz SR, Gregory TR, Mathieu J, Verdon L, Farinelli L, Schmutz J, Lindquist E, Feyereisen R, Van de Peer Y. The genome of Tetranychus urticae reveals herbivorous pest adaptations. Nature. 2011;479(7374):487–92.
Petre B, Lorrain C, Stukenbrock EH, Duplessis S. Host-specialized transcriptome of plant-associated organisms. Curr Opin Plant Biol. 2020;56:81–8.
Jonckheere W, Dermauw W, Zhurov V, Wybouw N, Van den Bulcke J, Villarroel CA, Greenhalgh R, Grbić M, Schuurink RC, Tirry L, Baggerman G, Clark RM, Kant MR, Vanholme B, Menschaert G, Van Leeuwen T. The salivary protein repertoire of the polyphagous spider mite Tetranychus urticae : a quest for effectors. Mol Cell Proteomic. 2016;15(12):3594–613.
Mathers TC, Chen Y, Kaithakottil G, Legeai F, Mugford ST, Baa-Puyoulet P, Bretaudeau A, Clavijo B, Colella S, Collin O, Dalmay T, Derrien T, Feng H, Gabaldón T, Jordan A, Julca I, Kettles GJ, Kowitwanich K, Lavenier D, Lenzi P, Lopez-Gomollon S, Loska D, Mapleson D, Maumus F, Moxon S, Price DRG, Sugio A, van Munster M, Uzest M, Waite D, Jander G, Tagu D, Wilson ACC, van Oosterhout C, Swarbreck D, Hogenhout SA. Rapid transcriptional plasticity of duplicated gene clusters enables a clonally reproducing aphid to colonise diverse plant species. Genome Biol. 2017;18(1):27.
Stafford-Banks CA, Rotenberg D. Johnson B,R., Whitfield AE, Ullman DE: Analysis of the salivary gland transcriptome of Frankliniella occidentalis . PLoS One. 2014;9(4):e94447.
Montero-Astúa M, Ullman DE, Whitfield AE. Salivary gland morphology, tissue tropism and the progression of tospovirus infection in Frankliniella occidentalis . Virology. 2016;493:39–51.
Li B, Dewey CN. RSEM: accurate transcript quantification from RNA-Seq data with or without a reference genome. BMC Bioinformatics. 2011;12:323.
Boonham N, Smith P, Walsh K, Tame J, Morris J, Spence N, Bennison J, Barker I. Detection of Tomato spotted wilt virus (TSWV) in individual thrips using real time fluorescent RT-PCR (Taqman). J Virol Meth. 2002;101(1–2):37–48.
Wang H, Reitz S, Wang L, Wang S, Li X, Lei Z. The mRNA expression profiles of five heat shock protein genes from Frankliniella occidentalis at different stages and their responses to temperatures and insecticides. J Integr Agric. 2014;13:2196–210.
Livak KJ, Schmittgen TD. Analysis of relative gene expression data using real-time quantitative PCR and the 2−ΔΔCT method. Methods. 2001;25(4):402–8.
Käll L, Krogh A, Sonnhammer EL. Advantages of combined transmembrane topology and signal peptide prediction--the Phobius web server. Nucleic Acids Res. 2007;35(Web Server issue):429.
Petersen TN, Brunak S, von Heijne G, Nielsen H. SignalP 4.0: discriminating signal peptides from transmembrane regions. Nat Methods. 2011;8(10):785–6.
Höglund A, Dönnes P, Blum T, Adolph HW, Kohlbacher O. MultiLoc: prediction of protein subcellular localization using N-terminal targeting sequences, sequence motifs and amino acid composition. Bioinformatics. 2006;22(10):1158–65.
Valenzuela JG, Francischetti IM, Pham VM, Garfield MK, Ribeiro JM. Exploring the salivary gland transcriptome and proteome of the Anopheles stephensi mosquito. Insect Biochem Mol Biol. 2003;33(7):717–32.
Aoki J, Inoue A, Makide K, Saiki N, Arai H. Structure and function of extracellular phospholipase A1 belonging to the pancreatic lipase gene family. Biochimie. 2007;89(2):197–204.
Hogenhout SA, Van der Hoorn RA, Terauchi R, Kamoun S. Emerging concepts in effector biology of plant-associated organisms. Mol Plant Microbe Interact. 2009;22(2):115–22.
Dörmann P. Galactolipids in plant membranes. eLS. 2013;10.1002/9780470015902.a0020100.pub2.
Feyereisen R. Insect P450 enzymes. Annu Rev Entomol. 1999;44(1):507–33.
Heidel-Fischer HM, Vogel H. Molecular mechanisms of insect adaptation to plant secondary compounds. Curr Opin Insect Sci. 2015;8:8–14.
Cifuentes D, Chynoweth R, Guillén J, De La Rúa P, Bielza P. Novel cytochrome P450 genes, CYP6EB1 and CYP6EC1, are over-expressed in acrinathrin-resistant Frankliniella occidentalis (Thysanoptera: Thripidae). J Econ Entomol. 2012;105(3):1006–18.
Yan DK, Hu M, Tang YX, Fan JQ. Proteomic analysis reveals resistance mechanism against chlorpyrifos in Frankliniella occidentalis (Thysanoptera: Thripidae). J Econ Entomol. 2015;108(4):2000–8.
Nelson DR. Cytochrome P450 nomenclature. Methods Mol Biol. 1998;107:15–24.
Chung H, Sztal T, Pasricha S, Sridhar M, Batterham P. Characterization of Drosophila melanogaster cytochrome P450 genes. Proc Natl Acad Sci U S A. 2009;106(14):5731–6.
Zhu F, Moural TW, Shah K, Palli SR. Integrated analysis of cytochrome P450 gene superfamily in the red flour beetle, Tribolium castaneum . BMC Genomics. 2013;14:174.
Schneweis DJ. Characterizing global gene expression and antiviral response in Frankliniella occidentalis infected with Tomato spotted wilt virus. Manhattan: Kansas State University; 2017.
Saitou N, Nei M. The neighbor-joining method: a new method for reconstructing phylogenetic trees. Mol Biol Evol. 1987;4(4):406–25.
Felsenstein J. Confidence limits on phylogenies: an approach using the bootstrap. Evolution. 1985;39(4):783–91.
Zuckerkandl E, Pauling L. Evolutionary divergence and convergence in proteins. In: Bryson V, Vogel HJ, editors. Evolving Genes and Proteins. New York: Academic Press; 1965. p. 97–166.
Kumar S, Stecher G, Li M, Knyaz C, Tamura K. MEGA X: Molecular evolutionary genetics analysis across computing platforms. Mol Biol Evol. 2018;35(6):1547–9.
Iga M, Kataoka H. Recent studies on insect hormone metabolic pathways mediated by cytochrome P450 enzymes. Biol Pharm Bull. 2012;35(6):838–43.
Guittard E, Blais C, Maria A, Parvy JP, Pasricha S, Lumb C, Lafont R, Daborn PJ, Dauphin-Villemant C. CYP18A1, a key enzyme of Drosophila steroid hormone inactivation, is essential for metamorphosis. Dev Biol. 2011;349(1):35–45.
Sztal T, Chung H, Berger S, Currie PD, Batterham P, Daborn PJ. A cytochrome p450 conserved in insects is involved in cuticle formation. PLoS One. 2012;7(5):e36544.
Scott JG, Wen Z. Cytochromes P450 of insects: the tip of the iceberg. Pest Manag Sci. 2001;57(10):958–67.
Claudianos C, Ranson H, Johnson RM, Biswas S, Schuler MA, Berenbaum MR, Feyereisen R, Oakeshott JG. A deficit of detoxification enzymes: pesticide sensitivity and environmental response in the honeybee. Insect Mol Biol. 2006;15(5):615–36.
Després L, David JP, Gallet C. The evolutionary ecology of insect resistance to plant chemicals. Trends Ecol Evol. 2007;22(6):298–307.
Oakeshott JG, Claudianos C, Campbell PM, Newcomb RD, Russell RJ. 5.10 - Biochemical Genetics and Genomics of Insect Esterases. In: Gilbert LI, editor. Comprehensive Molecular Insect Science. Amsterdam: Elsevier; 2005. p. 309–81.
Bryon A, Wybouw N, Dermauw W, Tirry L, Van Leeuwen T. Genome wide gene-expression analysis of facultative reproductive diapause in the two-spotted spider mite Tetranychus urticae . BMC Genomics. 2013;14(1):815.
de Castro E, Sigrist CJ, Gattiker A, Bulliard V, Langendijk-Genevaux PS, Gasteiger E, Bairoch A, Hulo N. ScanProsite: detection of PROSITE signature matches and ProRule-associated functional and structural residues in proteins. Nucleic Acids Res. 2006;34(Web Server issue):362.
Dermauw W, Wybouw N, Rombauts S, Menten B, Vontas J, Grbic M, Clark RM, Feyereisen R, Van Leeuwen T. A link between host plant adaptation and pesticide resistance in the polyphagous spider mite Tetranychus urticae . Proc Natl Acad Sci U S A. 2013;110(2):113.
Dermauw W, Van Leeuwen T. The ABC gene family in arthropods: comparative genomics and role in insecticide transport and resistance. Insect Biochem Mol Biol. 2014;45:89–110.
Dermauw W, Osborne EJ, Clark RM, Grbić M, Tirry L, Van Leeuwen T. A burst of ABC genes in the genome of the polyphagous spider mite Tetranychus urticae . BMC Genomics. 2013;14(1):317.
Hull JJ, Chaney K, Geib SM, Fabrick JA, Brent CS, Walsh D, Lavine LC. Transcriptome-based identification of ABC transporters in the western tarnished plant bug Lygus hesperus . PLoS One. 2014;9(11):e113046.
Katoh K, Misawa K, Kuma K, Miyata T. MAFFT: a novel method for rapid multiple sequence alignment based on fast Fourier transform. Nucleic Acids Res. 2002;30(14):3059–66.
Qi W, Ma X, He W, Chen W, Zou M, Gurr GM, Vasseur L, You M. Characterization and expression profiling of ATP-binding cassette transporter genes in the diamondback moth, Plutella xylostella (L.). BMC Genomics. 2016;17(1):760.
Sturm A, Cunningham P, Dean M. The ABC transporter gene family of Daphnia pulex . BMC Genomics. 2009;10:170.
Sun H, Pu J, Chen F, Wang J, Han Z. Multiple ATP-binding cassette transporters are involved in insecticide resistance in the small brown planthopper, Laodelphax striatellus . Insect Mol Biol. 2017;26(3):343–55.
Tamura K, Stecher G, Peterson D, Filipski A, Kumar S. MEGA6: Molecular Evolutionary Genetics Analysis version 6.0. Mol Biol Evol. 2013;30(12):2725–9.
Tian L, Song T, He R, Zeng Y, Xie W, Wu Q, Wang S, Zhou X, Zhang Y. Genome-wide analysis of ATP-binding cassette (ABC) transporters in the sweetpotato whitefly, Bemisia tabaci . BMC Genomics. 2017;18(1):330.
Xie W, Yang X, Chen C, Yang Z, Guo L, Wang D, Huang J, Zhang H, Wen Y, Zhao J, Wu Q, Wang S, Coates BS, Zhou X, Zhang Y. The invasive MED/Q Bemisia tabaci genome: a tale of gene loss and gene gain. BMC Genomics. 2018;19(1):68.
Schinkel AH, Jonker JW. Mammalian drug efflux transporters of the ATP binding cassette (ABC) family: an overview. Adv Drug Deliv Rev. 2012;64:138–53.
Chen W, Hasegawa DK, Kaur N, Kliot A, Pinheiro PV, Luan J, Stensmyr MC, Zheng Y, Liu W, Sun H, Xu Y, Luo Y, Kruse A, Yang X, Kontsedalov S, Lebedev G, Fisher TW, Nelson DR, Hunter WB, Brown JK, Jander G, Cilia M, Douglas AE, Ghanim M, Simmons AM, Wintermantel WM, Ling K, Fei Z. The draft genome of whitefly Bemisia tabaci MEAM1, a global crop pest, provides novel insights into virus transmission, host adaptation, and insecticide resistance. BMC Biol. 2016;14(1):110.
Ramsey JS, Rider DS, Walsh TK, De Vos M, Gordon KH, Ponnala L, Macmil SL, Roe BA, Jander G. Comparative analysis of detoxification enzymes in Acyrthosiphon pisum and Myzus persicae . Insect Mol Biol. 2010;19(Suppl 2):155–64.
Huchard E, Martinez M, Alout H, Douzery EJ, Lutfalla G, Berthomieu A, Berticat C, Raymond M, Weill M. Acetylcholinesterase genes within the Diptera: takeover and loss in true flies. Proc Biol Sci. 2006;273(1601):2595–604.
Jensen SE. Insecticide resistance in the Western flower thrips, Frankliniella occidentalis . Integr Pest Manag Rev. 2000;5(2):131–46.
Mackert A, do Nascimento AM, Bitondi MM, Hartfelder K, Simões ZL. Identification of a juvenile hormone esterase-like gene in the honey bee, Apis mellifera L.--expression analysis and functional assays. Comp Biochem Physiol B Biochem Mol Biol. 2008;150(1):33–44.
Oakeshott JG, Johnson RM, Berenbaum MR, Ranson H, Cristino AS, Claudianos C. Metabolic enzymes associated with xenobiotic and chemosensory responses in Nasonia vitripennis . Insect Mol Biol. 2010;19(Suppl 1):147–63.
Yu QY, Lu C, Li WL, Xiang ZH, Zhang Z. Annotation and expression of carboxylesterases in the silkworm, Bombyx mori . BMC Genomics. 2009;10:553.
Lemaitre B, Hoffmann J. The host defense of Drosophila melanogaster . Annu Rev Immunol. 2007;25:697–743.
Ligoxygakis P. Genetics of immune recognition and response in Drosophila host defense. Adv Genet. 2013;83:71–97.
Ganesan S, Aggarwal K, Paquette N, Silverman N. NF-κB/Rel proteins and the humoral immune responses of Drosophila melanogaster . Curr Top Microbiol Immunol. 2011;349:25–60.
Davis MM, Engström Y. Immune response in the barrier epithelia: lessons from the fruit fly Drosophila melanogaster . J Innate Immun. 2012;4(3):273–83.
Ferrandon D. The complementary facets of epithelial host defenses in the genetic model organism Drosophila melanogaster : from resistance to resilience. Curr Opin Immunol. 2013;25(1):59–70.
Vlisidou I, Wood W. Drosophila blood cells and their role in immune responses. FEBS J. 2015;282(8):1368–82.
Kingsolver MB, Huang Z, Hardy RW. Insect antiviral innate immunity: pathways, effectors, and connections. J Mol Biol. 2013;425(24):4921–36.
Mussabekova A, Daeffler L, Imler JL. Innate and intrinsic antiviral immunity in Drosophila. Cell Mol Life Sci. 2017;74(11):2039–54.
Arp AP, Hunter WB, Pelz-Stelinski K. Annotation of the Asian citrus psyllid genome reveals a reduced innate immune system. Front Physiol. 2016;7:570.
Zhang C, Zhang S, Xia J, Li F, Xia W, Liu S, Wang X. The immune strategy and stress response of the Mediterranean species of the Bemisia tabaci complex to an orally delivered bacterial pathogen. PLoS One. 2014;9(4):e94477.
Nishide Y, Kageyama D, Yokoi K, Jouraku A, Tanaka H, Futahashi R, Fukatsu T. Functional crosstalk across IMD and Toll pathways: insight into the evolution of incomplete immune cascades. Proc Biol Sci. 2019;286(1897):20182207.
Altincicek B, Gross J, Vilcinskas A. Wounding-mediated gene expression and accelerated viviparous reproduction of the pea aphid Acyrthosiphon pisum . Insect Mol Biol. 2008;17(6):711–6.
Leone P, Bischoff V, Kellenberger C, Hetru C, Royet J, Roussel A. Crystal structure of Drosophila PGRP-SD suggests binding to DAP-type but not lysine-type peptidoglycan. Mol Immunol. 2008;45(9):2521–30.
Leulier F, Parquet C, Pili-Floury S, Ryu JH, Caroff M, Lee WJ, Mengin-Lecreulx D, Lemaitre B. The Drosophila immune system detects bacteria through specific peptidoglycan recognition. Nat Immunol. 2003;4(5):478–84.
Park JW, Je BR, Piao S, Inamura S, Fujimoto Y, Fukase K, Kusumoto S, Soderhall K, Ha NC, Lee BL. A synthetic peptidoglycan fragment as a competitive inhibitor of the melanization cascade. J Biol Chem. 2006;281(12):7747–55.
Yokoi K, Koyama H, Minakuchi C, Tanaka T, Miura K. Antimicrobial peptide gene induction, involvement of Toll and IMD pathways and defense against bacteria in the red flour beetle, Tribolium castaneum . Results Immunol. 2012;2:72–82.
Yokoi K, Koyama H, Ito W, Minakuchi C, Tanaka T, Miura K. Involvement of NF-kappaB transcription factors in antimicrobial peptide gene induction in the red flour beetle, Tribolium castaneum . Dev Comp Immunol. 2012;38(2):342–51.
Haas B, Papanicolaou A: TransDecoder (find coding regions within transcripts). 2016. https://github.com/TransDecoder/TransDecoder/wiki .
Xu L, Dong Z, Fang L, Luo Y, Wei Z, Guo H, Zhang G, Gu YQ, Coleman-Derr D, Xia Q, Wang Y. OrthoVenn2: a web server for whole-genome comparison and annotation of orthologous clusters across multiple species. Nucleic Acids Res. 2019;47(W1):W52–8.
Badillo-Vargas IE, Chen Y, Martin KM, Rotenberg D, Whitfield AE. Discovery of novel thrips vector proteins that bind to the viral attachment protein of the plant Bunyavirus tomato spotted wilt virus. J Virol. 2019;93(21) https://doi.org/10.1128/JVI.00699-19 . Print 2019 Nov 1.
Gerardo N, Altincicek B, Anselme C, Atamian H, Barribeau S, de Vos M, Duncan E, Evans J, Gabaldon T, Ghanim M, Heddi A, Kaloshian I, Latorre A, Moya A, Nakabachi A, Parker B, Perez-Brocal V, Pignatelli M, Rahbe Y, Ramsey J, Spragg C, Tamames J, Tamarit D, Tamborindeguy C, Vincent-Monegat C, Vilcinskas A. Immunity and other defenses in pea aphids, Acyrthosiphon pisum . Genome Biol. 2010;11(2):R21.
Naitza S, Rosse C, Kappler C, Georgel P, Belvin M, Gubb D, Camonis J, Hoffmann JA, Reichhart JM. The Drosophila immune defense against gram-negative infection requires the death protein dFADD. Immunity. 2002;17(5):575–81.
Gangloff M, Gay NJ. MD-2: the Toll 'gatekeeper' in endotoxin signalling. Trends Biochem Sci. 2004;29(6):294–300.
Min G, Sarkar IN, Siddall ME. Salivary transcriptome of the North American medicinal leech, Macrobdella decora . J Parasitol. 2010;96(6):1211–21.
Kim YM, Park K, Choi K, Alvarez RA, Cummings RD, Cho M. Lectin from the manila clam Ruditapes philippinarum is induced upon infection with the protozoan parasite Perkinsus olseni. J Biol Chem. 2006;281(37):26854–64.
Kamhawi S, Ramalho-Ortigao M, Pham VM, Kumar S, Lawyer PG, Turco SJ, Barillas-Mury C, Sacks DL, Valenzuela JG. A role for insect galectins in parasite survival. Cell. 2004;119(3):329–41.
Chai Y, Zhu Q, Yu S, Zhao X, Wang J. A novel protein with a fibrinogen-like domain involved in the innate immune response of Marsupenaeus japonicus . Fish Shellfish Immunol. 2012;32(2):307–15.
Wang XG, Zhao Q, Christensen BM. Identification and characterization of the fibrinogen-like domain of fibrinogen-related proteins in the mosquito, Anopheles gambiae , and the fruitfly, Drosophila melanogaster , genomes. BMC Genomics. 2005;6:114.
Parker JS, Mizuguchi K, Gay NJ. A family of proteins related to Spatzle, the toll receptor ligand, are encoded in the Drosophila genome. Proteins Struct Funct Genet. 2001;45(1):71–80.
Soudi M, Zamocky M, Jakopitsch C, Furtmueller PG, Obinger C. Molecular evolution, structure, and function of peroxidasins. Chem Biodivers. 2012;9(9):1776–93.
Orme M, Meier P. Inhibitor of apoptosis proteins in Drosophila: gatekeepers of death. Apoptosis. 2009;14(8):950–60.
Christiaens O, Swevers L, Smagghe G. DsRNA degradation in the pea aphid ( Acyrthosiphon pisum ) associated with lack of response in RNAi feeding and injection assay. Peptides. 2014;53:307–14.
Swevers L, Vanden Broeck J, Smagghe G. The possible impact of persistent virus infection on the function of the RNAi machinery in insects: a hypothesis. Front Physiol. 2013;4:319.
Belliure B, Janssen A, Maris PC, Peters D, Sabelis MW. Herbivore arthropods benefit from vectoring plant viruses. Ecol Lett. 2005;8:70–9.
Maris PC, Joosten NN, Goldbach RW, Peters D. Tomato spotted wilt virus infection improves host suitability for its vector Frankliniella occidentalis . Phytopathology. 2004;94:706–11.
Rotenberg D, Whitfield AE. Analysis of expressed sequence tags from Frankliniella occidentalis , the western flower thrips. Insect Mol Biol. 2010;19:537–51.
Dowling D, Pauli T, Donath A, Meusemann K, Podsiadlowski L, Petersen M, Peters RS, Mayer C, Liu S, Zhou X, Misof B, Niehuis O. Phylogenetic origin and diversification of RNAi pathway genes in insects. Genome Biol Evol. 2016;8(12):3784–93.
Taning CNT, Andrade EC, Hunter WB, Christiaens O, Smagghe G. Asian citrus psyllid RNAi pathway – RNAi evidence. Sci Rep. 2016;6(1):38082.
Viet Dung N, Christiaens O, Le Van Bao D, De Vos S, MacRae TH, Smagghe G, Bossier P. Identification of RNAi-related genes and transgenerational efficiency of RNAi in Artemia franciscana . Aquaculture. 2019;501:285–92.
Fukunaga R, Zamore PD. Chapter two - loquacious, a Dicer partner protein, functions in both the microRNA and siRNA pathways. Enzymes. 2012;32:37–68.
Haac ME, Anderson MA, Eggleston H, Myles KM, Adelman ZN. The hub protein loquacious connects the microRNA and short interfering RNA pathways in mosquitoes. Nucleic Acids Res. 2015;43(7):3688–700.
Murat S, Hopfen C, McGregor AP. The function and evolution of Wnt genes in arthropods. Arthropod Struct Dev. 2010;39(6):446–52.
Oberhofer G, Grossmann D, Siemanowski JL, Beissbarth T, Bucher G. Wnt/beta-catenin signaling integrates patterning and metabolism of the insect growth zone. Development. 2014;141(24):4740–50.
Bolognesi R, Farzana L, Fischer TD, Brown SJ. Multiple Wnt genes are required for segmentation in the short-germ embryo of Tribolium castaneum . Curr Biol. 2008;18(20):1624–9.
Janssen R, Schönauer A, Weber M, Turetzek N, Hogvall M, Goss G, Patel N, McGregor A, Hilbrant M. The evolution and expression of panarthropod frizzled genes. Front Ecol Evol. 2015;3:96.
Sullivan JC, Ryan JF, Mullikin JC, Finnerty JR. Conserved and novel Wnt clusters in the basal eumetazoan Nematostella vectensis . Dev Genes Evol. 2007;217(3):235–9.
Beermann A, Prühs R, Lutz R, Schröder R. A context-dependent combination of Wnt receptors controls axis elongation and leg development in a short germ insect. Development. 2011;138(13):2793–805.
Janssen R, Le Gouar M, Pechmann M, Poulin F, Bolognesi R, Schwager EE, Hopfen C, Colbourne JK, Budd GE, Brown SJ, Prpic NM, Kosiol C, Vervoort M, Damen WG, Balavoine G, McGregor AP. Conservation, loss, and redeployment of Wnt ligands in protostomes: implications for understanding the evolution of segment formation. BMC Evol Biol. 2010;10:374.
Boulanger A, Clouet-Redt C, Farge M, Flandre A, Guignard T, Fernando C, Juge F, Dura JM. ftz-f1 and Hr39 opposing roles on EcR expression during Drosophila mushroom body neuron remodeling. Nat Neurosci. 2011;14(1):37–44.
Shigenobu S, Richards S, Cree AG, Morioka M, Fukatsu T, Kudo T, Miyagishima S, Gibbs RA, Stern DL, Nakabachi A. A full-length cDNA resource for the pea aphid, Acyrthosiphon pisum . Insect Mol Biol. 2010;19(Suppl 2):23–31.
Nicholson SJ, Nickerson ML, Dean M, Song Y, Hoyt PR, Rhee H, Kim C, Puterka GJ. The genome of Diuraphis noxia , a global aphid pest of small grains. BMC Genomics. 2015;16(1):429.
Supek F, Bošnjak M, Škunca N, Šmuc T. REVIGO summarizes and visualizes long lists of gene ontology terms. PLoS One. 2011;6(7):e21800.
Belles X, Santos CG. The MEKRE93 (Methoprene tolerant-Kruppel homolog 1-E93) pathway in the regulation of insect metamorphosis, and the homology of the pupal stage. Insect Biochem Mol Biol. 2014;52:60–8.
Minakuchi C, Tanaka M, Miura K, Tanaka T. Developmental profile and hormonal regulation of the transcription factors broad and Kruppel homolog 1 in hemimetabolous thrips. Insect Biochem Mol Biol. 2011;41(2):125–34.
Truman JW, Riddiford LM. The evolution of insect metamorphosis: a developmental and endocrine view. Philos Trans R Soc Lond B Biol Sci. 2019;374(1783):20190070.
Zhu J, Chen L, Raikhel AS. Distinct roles of Broad isoforms in regulation of the 20-hydroxyecdysone effector gene, Vitellogenin, in the mosquito Aedes aegypti . Mol Cell Endocrinol. 2007;267(1–2):97–105.
Zhou X, Riddiford LM. rosy function is required for juvenile hormone effects in Drosophila melanogaster . Genetics. 2008;178(1):273–81.
Li M, Mead EA, Zhu J. Heterodimer of two bHLH-PAS proteins mediates juvenile hormone-induced gene expression. Proc Natl Acad Sci U S A. 2011;108(2):638–43.
Zhang Z, Xu J, Sheng Z, Sui Y, Palli SR. Steroid receptor co-activator is required for juvenile hormone signal transduction through a bHLH-PAS transcription factor, methoprene tolerant. J Biol Chem. 2011;286(10):8437–47.
Byun PK, Zhang C, Yao B, Wardwell-Ozgo J, Terry D, Jin P, Moberg K. The Taiman transcriptional coactivator engages Toll signals to promote apoptosis and intertissue invasion in Drosophila. Curr Biol. 2019;29(17):2790–2800.e4.
Zhu J, Chen L, Sun G, Raikhel AS. The competence factor beta Ftz-F1 potentiates ecdysone receptor activity via recruiting a p160/SRC coactivator. Mol Cell Biol. 2006;26(24):9402–12.
Bialecki M, Shilton A, Fichtenberg C, Segraves WA, Thummel CS. Loss of the ecdysteroid-inducible E75A orphan nuclear receptor uncouples molting from metamorphosis in Drosophila. Dev Cell. 2002;3(2):209–20.
Bernardo TJ, Dubrovsky EB. Molecular mechanisms of transcription activation by juvenile hormone: A critical role for bHLH-PAS and nuclear receptor proteins. Insects. 2012;3(1):324–38.
Rewitz KF, Yamanaka N, O'Connor MB. Steroid hormone inactivation is required during the juvenile-adult transition in Drosophila. Developmental cell. 2010;19(6):895–902.
Li Z, Ge X, Ling L, Zeng B, Xu J, Aslam AFM, You L, Palli SR, Huang Y, Tan A. CYP18A1 regulates tissue-specific steroid hormone inactivation in Bombyx mori . Insect Biochem Mol Biol. 2014;54:33–41.
Tarver MR, Coy MR, Scharf ME. Cyp15F1: a novel cytochrome P450 gene linked to juvenile hormone-dependent caste differention in the termite Reticulitermes flavipes . Arch Insect Biochem Physiol. 2012;80(2):92–108.
Crone EJ, Sutherland TD, Campbell PM, Coppin CW, Russell RJ, Oakeshott JG. Only one esterase of Drosophila melanogaster is likely to degrade juvenile hormone in vivo. Insect Biochem Mol Biol. 2007;37(6):540–9.
Blair SS, Brower DL, Thomas JB, Zavortink M. The role of apterous in the control of dorsoventral compartmentalization and PS integrin gene expression in the developing wing of Drosophila. Development. 1994;120(7):1805–15.
Willis JH. Structural cuticular proteins from arthropods: annotation, nomenclature, and sequence characteristics in the genomics era. Insect Biochem Mol Biol. 2010;40(3):189–204.
Ioannidou ZS, Theodoropoulou MC, Papandreou NC, Willis JH, Hamodrakas SJ. CutProtFam-Pred: detection and classification of putative structural cuticular proteins from sequence alone, based on profile hidden Markov models. Insect Biochem Mol Biol. 2014;52:51–9.
Fahrbach SE, Smagghe G, Velarde RA. Insect nuclear receptors. Annu Rev Entomol. 2012;57:83–106.
Gilbert L. Insect development: Morphogenesis, molting and metamorphosis. Oxford: Academic Press; 2009.
Christiaens O, Iga M, Velarde RA, Rouge P, Smagghe G. Halloween genes and nuclear receptors in ecdysteroid biosynthesis and signalling in the pea aphid. Insect Mol Biol. 2010;19(Suppl 2):187–200.
Scolari F, Gomulski LM, Ribeiro JMC, Siciliano P, Meraldi A, Falchetto M, Bonomi A, Manni M, Gabrieli P, Malovini A, Bellazzi R, Aksoy S, Gasperi G, Malacrida AR. Transcriptional profiles of mating-responsive genes from testes and male accessory glands of the Mediterranean fruit fly, Ceratitis capitata . PLoS One. 2012;7(10):e46812.
Bridges M, Jones AME, Bones AM, Hodgson C, Cole R, Bartlet E, Wallsgrove R, Karapapa VK, Watts N, Rossiter JT. Spatial organization of the glucosinolate-myrosinase system in brassica specialist aphids is similar to that of the host plant. Proc Biol Sci. 2002;269(1487):187–91.
Dawson GW, Griffiths DC, Pickett JA, Wadhams LJ, Woodcock CM. Plant-derived synergists of alarm pheromone from turnip aphid, Lipaphis (Hyadaphis) erysimi (Homoptera, Aphididae). J Chem Ecol. 1987;13(7):1663–71.
Thomas GWC, Dohmen E, Hughes DST, Murali SC, Poelchau M, Glastad K, Anstead CA, Ayoub NA, Batterham P, Bellair M, Binford GJ, Chao H, Chen YH, Childers C, Dinh H, Doddapaneni HV, Duan JJ, Dugan S, Esposito LA, Friedrich M, Garb J, Gasser RB, Goodisman MAD, Gundersen-Rindal D, Han Y, Handler AM, Hatakeyama M, Hering L, Hunter WB, Ioannidis P, Jayaseelan JC, Kalra D, Khila A, Korhonen PK, Lee CE, Lee SL, Li Y, Lindsey ARI, Mayer G, McGregor AP, McKenna DD, Misof B, Munidasa M, Munoz-Torres M, Muzny DM, Niehuis O, Osuji-Lacy N, Palli SR, Panfilio KA, Pechmann M, Perry T, Peters RS, Poynton HC, Prpic N, Qu J, Rotenberg D, Schal C, Schoville SD, Scully ED, Skinner E, Sloan DB, Stouthamer R, Strand MR, Szucsich NU, Wijeratne A, Young ND, Zattara EE, Benoit JB, Zdobnov EM, Pfrender ME, Hackett KJ, Werren JH, Worley KC, Gibbs RA, Chipman AD, Waterhouse RM, Bornberg-Bauer E, Hahn MW, Richards S. Gene content evolution in the arthropods. Genome Biol. 2020;21(1):15.
Bautista RC, Mau RFL, Cho JJ, Custer DM. Potential of Tomato spotted wilt tospovirus plant hosts in Hawaii as virus reservoirs for transmission by Frankliniella occidentalis (Thysanoptera:Thripidae). Phytopathology. 1995;85:953–8.
Gnerre S, Maccallum I, Przybylski D, Ribeiro FJ, Burton JN, Walker BJ, Sharpe T, Hall G, Shea TP, Sykes S, Berlin AM, Aird D, Costello M, Daza R, Williams L, Nicol R, Gnirke A, Nusbaum C, Lander ES, Jaffe DB. High-quality draft assemblies of mammalian genomes from massively parallel sequence data. Proc Natl Acad Sci U S A. 2011;108(4):1513–8.
Atlas-Link: https://www.hgsc.bcm.edu/software/atlas-link . [ https://www.hgsc.bcm.edu/software/atlas-link ].
ATLAS gapfill 2.2: https://www.hgsc.bcm.edu/software/atlas-gapfill . https://www.hgsc.bcm.edu/software/atlas-gapfill .
Cantarel BL, Korf I, Robb SM, Parra G, Ross E, Moore B, Holt C, Sanchez Alvarado A, Yandell M. MAKER: an easy-to-use annotation pipeline designed for emerging model organism genomes. Genome Res. 2008;18(1):188–96.
Stanke M, Diekhans M, Baertsch R, Haussler D. Using native and syntenically mapped cDNA alignments to improve de novo gene finding. Bioinformatics. 2008;24(5):637–44.
Korf I. Gene finding in novel genomes. BMC Bioinformatics. 2004;5:59.
Baylor College of Medicine – Human Genome Sequencing Center, Western Flower Thrips Genome Project: https://www.hgsc.bcm.edu/arthropods/western-flower-thrips-genome-project .
Frankliniella occidentalis i5k Workspace@NAL (National Agricultural Library): https://i5k.nal.usda.gov/Frankliniella_occidentalis . Frankliniella occidentalis i5k Workspace@NAL (National Agricultural Library).
Goff SA, Vaughn M, McKay S, Lyons E, Stapleton AE, Gessler D, Matasci N, Wang L, Hanlon M, Lenards A, Muir A, Merchant N, Lowry S, Mock S, Helmke M, Kubach A, Narro M, Hopkins N, Micklos D, Hilgert U, Gonzales M, Jordan C, Skidmore E, Dooley R, Cazes J, McLay R, Lu Z, Pasternak S, Koesterke L, Piel WH, Grene R, Noutsos C, Gendler K, Feng X, Tang C, Lent M, Kim S, Kvilekval K, Manjunath BS, Tannen V, Stamatakis A, Sanderson M, Welch SM, Cranston KA, Soltis P, Soltis D, O'Meara B, Ane C, Brutnell T, Kleibenstein DJ, White JW, Leebens-Mack J, Donoghue MJ, Spalding EP, Vision TJ, Myers CR, Lowenthal D, Enquist BJ, Boyle B, Akoglu A, Andrews G, Ram S, Ware D, Stein L, Stanzione D. The iPlant Collaborative: Cyberinfrastructure for plant biology. Front Plant Sci. 2011;2:34.
Schmieder R, Edwards R. Quality control and preprocessing of metagenomic datasets. Bioinformatics. 2011;27(6):863–4.
Wu TD, Watanabe CK. GMAP: a genomic mapping and alignment program for mRNA and EST sequences. Bioinformatics. 2005;21(9):1859–75.
Kriventseva EV, Tegenfeldt F, Petty TJ, Waterhouse RM, Simão FA, Pozdnyakov IA, Ioannidis P, Zdobnov EM. OrthoDB v8: update of the hierarchical catalog of orthologs and the underlying free software. Nucleic Acids Res. 2015;43:D250–6.
Stamatakis A. RAxML-VI-HPC: maximum likelihood-based phylogenetic analyses with thousands of taxa and mixed models. Bioinformatics. 2006;22(21):2688–90.
Edgar RC. MUSCLE: a multiple sequence alignment method with reduced time and space complexity. BMC Bioinformatics. 2004;5(1):113.
Rice P, Longden I, Bleasby A. EMBOSS: the European Molecular Biology Open Software Suite. Trends Genet. 2000;16(6):276–7.
Simao FA, Waterhouse RM, Ioannidis P, Kriventseva EV, Zdobnov EM. BUSCO: assessing genome assembly and annotation completeness with single-copy orthologs. Bioinformatics. 2015;31(19):3210–2.
Kajitani R, Toshimoto K, Noguchi H, Toyoda A, Ogura Y, Okuno M, Yabana M, Harada M, Nagayasu E, Maruyama H, Kohara Y, Fujiyama A, Hayashi T, Itoh T. Efficient de novo assembly of highly heterozygous genomes from whole-genome shotgun short reads. Genome Res. 2014;24(8):1384–95.
Pryszcz LP, Gabaldón T. Redundans: an assembly pipeline for highly heterozygous genomes. Nucleic Acids Res. 2016;44(12):e113.
Weirauch MT, Yang A, Albu M, Cote AG, Montenegro-Montero A, Drewe P, Najafabadi HS, Lambert SA, Mann I, Cook K, Zheng H, Goity A, van Bakel H, Lozano JC, Galli M, Lewsey MG, Huang E, Mukherjee T, Chen X, Reece-Hoyes JS, Govindarajan S, Shaulsky G, Walhout AJM, Bouget FY, Ratsch G, Larrondo LF, Ecker JR, Hughes TR. Determination and inference of eukaryotic transcription factor sequence specificity. Cell. 2014;158(6):1431–43.
Finn RD, Mistry J, Tate J, Coggill P, Heger A, Pollington JE, Gavin OL, Gunasekaran P, Ceric G, Forslund K, Holm L, Sonnhammer ELL, Eddy SR, Bateman A. The Pfam protein families database. Nucleic Acids Res. 2010;38(suppl_1):D211–22.
Weirauch MT, Hughes TR. A catalogue of eukaryotic transcription factor types, their evolutionary origin, and species distribution. In: Hughes T, editor. A Handbook of Transcription Factors, vol. 52. Dordrecht: Springer; 2011. p. 25–73.
Eddy SR. A new generation of homology search tools based on probabilistic inference. Genome Inform. 2009;23(1):205–1.
PubMed Google Scholar
Morgulis A, Gertz EM, Schäffer AA, Agarwala R. A fast and symmetric DUST implementation to mask low-complexity DNA sequences. J Comput Biol. 2006;13(5):1028–40.
Telleria EL, Benoit JB, Zhao X, Savage AF, Regmi S, Alves e Silva TL, O'Neill M, Aksoy S. Insights into the trypanosome-host interactions revealed through transcriptomic analysis of parasitized tsetse fly salivary glands. PLoS Negl Trop Dis. 2014;8(4):e2649.
Ribeiro JM, Martin-Martin I, Arcà B, Calvo E. A deep insight into the sialome of male and female Aedes aegypti mosquitoes. PLoS One. 2016;11(3):e0151400.
Baggerly KA, Deng L, Morris JS, Aldaz CM. Differential expression in SAGE: accounting for normal between-library variation. Bioinformatics. 2003;19(12):1477–83.
Benjamini Y, Hochberg Y. Controlling the false discovery rate: a practical and powerful approach to multiple testing. J R Statist Soc B. 1995;57(1):289–300.
Almagro Armenteros JJ, Tsirigos KD, Sønderby CK, Petersen TN, Winther O, Brunak S, von Heijne G, Nielsen H. SignalP 5.0 improves signal peptide predictions using deep neural networks. Nat Biotechnol. 2019;37(4):420–3.
Krogh A, Larsson B, von Heijne G, Sonnhammer ELL. Predicting transmembrane protein topology with a hidden markov model: application to complete genomes11Edited by F. Cohen. J Mol Biol. 2001;305(3):567–80.
Almagro Armenteros JJ, Sønderby CK, Sønderby SK, Nielsen H, Winther O. DeepLoc: prediction of protein subcellular localization using deep learning. Bioinformatics. 2017;33(21):3387–95.
Waterhouse RM, Kriventseva EV, Meister S, Xi Z, Alvarez KS, Bartholomay LC, Barillas-Mury C, Bian G, Blandin S, Christensen BM, Dong Y, Jiang H, Kanost MR, Koutsos AC, Levashina EA, Li J, Ligoxygakis P, MacCallum RM, Mayhew GF, Mendes A, Michel K, Osta MA, Paskewitz S, Shin SW, Vlachou D, Wang L, Wei W, Zheng L, Zou Z, Severson DW, Raikhel AS, Kafatos FC, Dimopoulos G, Zdobnov EM, Christophides GK. Evolutionary dynamics of immune-related genes and pathways in disease-vector mosquitoes. Science. 2007;316(5832):1738–43.
Scolari F, Benoit JB, Michalkova V, Aksoy E, Takac P, Abd-Alla AMM, Malacrida AR, Aksoy S, Attardo GM. The spermatophore in Glossina morsitans morsitans : insights into male contributions to reproduction. Sci Rep. 2016;6(1):20334.
Schoville SD, Chen YH, Andersson MN, Benoit JB, Bhandari A, Bowsher JH, Brevik K, Cappelle K, Chen MM, Childers AK, Childers C, Christiaens O, Clements J, Didion EM, Elpidina EN, Engsontia P, Friedrich M, García-Robles I, Gibbs RA, Goswami C, Grapputo A, Gruden K, Grynberg M, Henrissat B, Jennings EC, Jones JW, Kalsi M, Khan SA, Kumar A, Li F, Lombard V, Ma X, Martynov A, Miller NJ, Mitchell RF, Munoz-Torres M, Muszewska A, Oppert B, Palli SR, Panfilio KA, Pauchet Y, Perkin LC, Petek M, Poelchau MF, Record É, Rinehart JP, Robertson HM, Rosendale AJ, Ruiz-Arroyo VM, Smagghe G, Szendrei Z, Thomas GWC, Torson AS, Vargas Jentzsch IM, Weirauch MT, Yates AD, Yocum GD, Yoon JS, Richards S. A model species for agricultural pest genomics: the genome of the Colorado potato beetle, Leptinotarsa decemlineata (Coleoptera: Chrysomelidae). Sci Rep. 2018;8(1):1931.
Bioinformatics & Evolutionary Genomics: Calculate and draw custom Venn diagrams: http://bioinformatics.psb.ugent.be/webtools/Venn/ .
Hughes DST, Richards S, Rotenberg D: Frankliniella occidentalis genome annotations v0.5.3. Ag Data Commons (Database). 2019. https://doi.org/10.15482/USDA.ADC/1503959 .
Rotenberg D, Gibbs RA, Worley KC, Murali SC, Lee SL, Muzny DM, Hughes DST, Chao H, Dinh H, Doddapaneni H, Qu J, Dugan-Perez S, Han Y, Richards S: Frankliniella occidentalis genome assembly v1.0. Ag Data Commons (Database). 2019. https://doi.org/10.15482/USDA.ADC/1503960 .
Rotenberg D, Robertson HM, Oliver JE, Benoit JB, Snoeck S, Baumann AA, Ben-Mahmoud S, Veenstra JA, Jacobs CGC, Park Y, Panfilio KA, Widana Gamage, S. M. K., Ahn SJ, Vargas Jentzsch IM, Jennings EC, Szuter EM, Christiaens O, Scanlan J, Taning CNT, Martynov A, Bejerman NE, Nair A, Jones JW, Friedrich M, van der Zee M, Dearden P, Minakuchi C, Armisén D, Schneweis DJ, Melo FL, Poelchau M, Dermauw W, Hughes DST, Richards S, Didion EM, Holmes CJ, Rosendale AJ, Rosselot A, Dolan A, Whitfield AE, Ullman DE, Dietzgen RG, Smagghe G, Van Leeuwen T, Werren JH: Frankliniella occidentalis Official Gene Set OGSv1.0. Ag Data Commons (Database). 2019. https://doi.org/10.15482/USDA.ADC/1504029 .
Download references
Acknowledgements
We thank the staff at the Baylor College of Medicine Human Genome Sequencing Center for their contributions. We thank David Nelson (University of Tennessee, USA) for officially naming the CYP sequences generated from this study. We also thank undergraduate students Nathan Nager, Michael Cosmos, and William Crusey for assisting HMR with analysis of the chemoreceptor gene families. We thank Anita Shrestha (University of Georgia, USA, currently Vertex Pharmaceuticals, USA) and Rajagopalbab (Babu) Srinivasan (University of Georgia, USA) for sharing the de novo-assembled transcriptome sequences of Frankliniella fusca from a previously published study for use in this study. We also thank Eileen Rendahl for graphic design of Fig. 1 .
Funding for genome sequencing, assembly, and automated annotation was provided by NHGRI grant U54 HG003273 to RA Gibbs. We also acknowledge NSF grants DEB1257053 and IOS1456233 to JH Werren, University of Cincinnati Faculty Development Research grant and NSF grant DEB 1654417 to JB Benoit, and Swiss NSF awards 31003A-125350 and 31003A-143936 to EM Zdobnov. RM Waterhouse was supported by Swiss National Science Foundation grant PP00P3_170664. S Snoeck, W Dermauw, and T Van Leeuwen were supported by Research Foundation Flanders (FWO) grant (G053815N). DE Ullman, D Rotenberg, AE Whitfield, JE Oliver, S Ben-Mahmoud, and DJ Schneweis were supported by AFRI NIFA Coordinated Agricultural Project grant (2012-68004-20166). DE Ullman, D Rotenberg, JB Benoit, S Ben-Mahmoud, and SP Rajarapu were supported by USDA NIFA grant (2018-67013-28495). The funders had no role in study design, data collection and analysis, decision to publish, or preparation of the manuscript.
Author information
Authors and affiliations.
Department of Entomology and Plant Pathology, North Carolina State University, Raleigh, NC, 27695, USA
Dorith Rotenberg, Swapna Priya Rajarapu & Anna E. Whitfield
Virology Section, College of Veterinary Medicine, University of Tennessee, A239 VTH, 2407 River Drive, Knoxville, TN, 37996, USA
Aaron A. Baumann
Department of Entomology and Nematology, University of California Davis, Davis, CA, 95616, USA
Sulley Ben-Mahmoud & Diane E. Ullman
Laboratory of Agrozoology, Department of Plants and Crops, Ghent University, Coupure Links 653, 9000, Ghent, Belgium
Olivier Christiaens, Wannes Dermauw, Simon Snoeck, Clauvis N. T. Taning, Dong Wei, Guy Smagghe & Thomas Van Leeuwen
Institute of Molecular Biology and Biotechnology, Foundation for Research and Technology-Hellas, Vassilika Vouton, 70013, Heraklion, Greece
Panagiotis Ioannidis
Department of Genetic Medicine and Development, University of Geneva Medical School, and Swiss Institute of Bioinformatics, Geneva, Switzerland
Panagiotis Ioannidis, Felipe A. Simão & Evgeny M. Zdobnov
Institute of Biology, Leiden University, 2333 BE, Leiden, The Netherlands
Chris G. C. Jacobs & Maurijn van der Zee
Institute for Zoology: Developmental Biology, University of Cologne, 50674, Cologne, Germany
Iris M. Vargas Jentzsch & Kristen A. Panfilio
Department of Plant Pathology, University of Georgia - Tifton Campus, Tifton, GA, 31793-5737, USA
Jonathan E. Oliver
National Agricultural Library, USDA-ARS, Beltsville, MD, 20705, USA
Monica F. Poelchau & Christopher Childers
Department of Plant Pathology, Kansas State University, Manhattan, KS, 66506, USA
Derek J. Schneweis, Kaylee Hervey & Brandi A. Schneweis
Department of Biology, University of Washington, Seattle, WA, 98105, USA
Simon Snoeck
Chongqing Key Laboratory of Entomology and Pest Control Engineering, College of Plant Protection, Southwest University, Chongqing, China
Dong Wei & Guy Smagghe
International Joint Laboratory of China-Belgium on Sustainable Crop Pest Control, Academy of Agricultural Sciences, Southwest University, Chongqing, China and Ghent University, Ghent, Belgium
Department of Botany, University of Ruhuna, Matara, Sri Lanka
Shirani M. K. Widana Gamage
Human Genome Sequencing Center, Department of Human and Molecular Genetics, Baylor College of Medicine, One Baylor Plaza, Houston, TX, 77030, USA
Daniel S. T. Hughes, Shwetha C. Murali, Hsu Chao, Huyen Dinh, Harsha Vardhan Doddapaneni, Shannon Dugan, Yi Han, Sandra L. Lee, Donna M. Muzny, Jiaxin Qu, Kim C. Worley, Richard A. Gibbs & Stephen Richards
Department of Biological Sciences, University of Cincinnati, Cincinnati, OH, 45221, USA
Samuel T. Bailey, Christopher J. Holmes, Emily C. Jennings, Andrew J. Rosendale, Andrew Rosselot & Joshua B. Benoit
IPAVE-CIAP-INTA, 5020, Cordoba, Argentina
Nicolas E. Bejerman
Department of Biology, Mount St. Joseph University, Cincinnati, OH, 45233, USA
Andrew J. Rosendale
Department of Biology, University of Rochester, Rochester, NY, 14627, USA
Sammy Cheng & John H. Werren
Queensland Alliance for Agriculture and Food Innovation, The University of Queensland, St. Lucia, QLD, 4072, Australia
Ralf G. Dietzgen
Department of Biological Sciences, Wayne State University, Detroit, MI, 48202, USA
Markus Friedrich & Jeffery W. Jones
School of Life Sciences, University of Warwick, Gibbet Hill Campus, Coventry, CV4 7AL, UK
Kristen A. Panfilio
Department of Entomology, Kansas State University, Manhattan, KS, 66506, USA
Yoonseong Park
Department of Entomology, University of Illinois at Urbana-Champaign, Urbana, IL, 61801, USA
Hugh M. Robertson
INCIA UMR 5287 CNRS, University of Bordeaux, Pessac, France
Jan A. Veenstra
Department of Ecology and Evolution, Swiss Institute of Bioinformatics, University of Lausanne, 1015, Lausanne, Switzerland
Robert M. Waterhouse
Center for Autoimmune Genomics and Etiology, Divisions of Biomedical Informatics and Developmental Biology, Cincinnati Children’s Hospital Medical Center, Cincinnati, OH, 45229, USA
Matthew T. Weirauch
Department of Pediatrics, University of Cincinnati, College of Medicine, Cincinnati, OH, 45229, USA
You can also search for this author in PubMed Google Scholar
Contributions
SR and DR conceived the project. DR coordinated the project. DR and AEW provided the biological material for sequencing, KH and BAS produced the sib-sib inbred line and extracted DNA for sequencing, and DJS extracted RNA for sequencing. SR, DMM, and RAG served key roles in program management of the sequencing project. SD, SLL, HC, HVD, HD, YH, and DMM constructed the libraries and sequenced the genome. SR, JQ, SCM, DSTH, and KCW assembled the genome and performed the automated gene predictions. MFP and CC implemented the Web Apollo-based manual curation. JHW and SC performed the bacterial scaffold detection and LGT analysis, and CNTT and DW performed PCR validation of LGTs. CGCJ, MvdZ, DJS, SMKW-G, NEB, ECJ, AJR, AR, AAB, OC, IMVJ, SB-M, JBB, MTW, JEO, SS, CNTT, WD, MF, JWJ, KAP, YP, HMR, JAV, GS, TVL, DEU, AEW, and RGD participated in manually curating genes and/or supervised curators. MFP performed quality control of the manual curation and provided the official gene set. PI, RMW, FAS and EMZ performed the analyses of gene set completeness and genome assembly quality. KAP and IMVJ analyzed homeodomain transcription factor gene structure and cluster synteny to assess assembly quality. MTW performed the genome-wide analysis of transcription factors. SB and JBB performed WGCNA of development with contributions from DR and DJS. JBB performed comparative analyses of RNA-seq datasets to generate the salivary gland gene set and SB-M performed real-time qRT-PCR to validate the SG analysis. SPR data-mined transcriptomes for innate immune gene transcripts for comparative analysis. CJH and JBB performed comparative analyses of sex-specific genes. DR prepared the manuscript and AAB, SB-M, JBB, SC, OC, WD, MF, PI, CGCJ, ECJ, IMVJ, JWJ, JEO, KAP, YP, SR, HMR, AJR, AR, DR, GS, SS, CNTT, DEU, TVL, JAV, RMW, MTW, JHW, and AEW contributed to supplemental notes, including phylogenetic trees, supplementary tables, and writing of the manuscript. DR and SPR prepared the supplementary notes and DR assembled the large supplementary tables. DEU, MTW, JBB, PI, and SPR prepared primary figures. All authors read and approved the final manuscript.
Corresponding author
Correspondence to Dorith Rotenberg .
Ethics declarations
Ethics approval and consent to participate.
Not applicable
Competing interests
The authors declare they have no competing interests.
Additional information
Publisher’s note.
Springer Nature remains neutral with regard to jurisdictional claims in published maps and institutional affiliations.
The original version of this article was revised: the phrase ‘vertically transmitted’ has been removed from the last sentence in the fourth paragraph under the heading 'Background'.
Supplementary information
Additional file 1..
Supplementary notes, figures and small tables.
Additional file 2.
Workbook of large supplementary tables.
Additional file 3.
Weighted correlation network analysis (WGCNA) modules of co-expressed gene sets of developmental stages.
Additional file 4.
Chemosensory receptor sequences.
Additional file 5.
Neuropeptide and protein hormone peptide sequences with highlighted features.
Additional file 6.
Complete and partial cytochrome p450 (CYP) sequences.
Additional file 7.
Complete ATP-binding cassette transporter (ABC) and carboxylesterase sequences.
Additional file 8.
Supporting tables for Orthovenn comparison of innate immunity-associated transcripts in thrips vector species.
Additional file 9.
Supporting tables of RNAseq expression outputs for conserved sex-specific genes in thrips species.
Additional file 10.
Custom Perl scripts used to compute the number of genes in each category of the phylogenomic analysis of the official gene set shown in Fig. 2 a.
Rights and permissions
Open Access This article is licensed under a Creative Commons Attribution 4.0 International License, which permits use, sharing, adaptation, distribution and reproduction in any medium or format, as long as you give appropriate credit to the original author(s) and the source, provide a link to the Creative Commons licence, and indicate if changes were made. The images or other third party material in this article are included in the article's Creative Commons licence, unless indicated otherwise in a credit line to the material. If material is not included in the article's Creative Commons licence and your intended use is not permitted by statutory regulation or exceeds the permitted use, you will need to obtain permission directly from the copyright holder. To view a copy of this licence, visit http://creativecommons.org/licenses/by/4.0/ . The Creative Commons Public Domain Dedication waiver ( http://creativecommons.org/publicdomain/zero/1.0/ ) applies to the data made available in this article, unless otherwise stated in a credit line to the data.
Reprints and permissions
About this article
Cite this article.
Rotenberg, D., Baumann, A.A., Ben-Mahmoud, S. et al. Genome-enabled insights into the biology of thrips as crop pests. BMC Biol 18 , 142 (2020). https://doi.org/10.1186/s12915-020-00862-9
Download citation
Received : 18 February 2020
Accepted : 02 September 2020
Published : 19 October 2020
DOI : https://doi.org/10.1186/s12915-020-00862-9
Share this article
Anyone you share the following link with will be able to read this content:
Sorry, a shareable link is not currently available for this article.
Provided by the Springer Nature SharedIt content-sharing initiative
- Thysanoptera
- Western flower thrips
- Hemipteroid assemblage
- Insect genomics
- Salivary glands
BMC Biology
ISSN: 1741-7007
- Submission enquiries: [email protected]
- General enquiries: [email protected]

An official website of the United States government
The .gov means it’s official. Federal government websites often end in .gov or .mil. Before sharing sensitive information, make sure you’re on a federal government site.
The site is secure. The https:// ensures that you are connecting to the official website and that any information you provide is encrypted and transmitted securely.
- Publications
- Account settings
Preview improvements coming to the PMC website in October 2024. Learn More or Try it out now .
- Advanced Search
- Journal List
- Wiley Open Access Collection

A global invasion by the thrip, Frankliniella occidentalis : Current virus vector status and its management
1 State Key Laboratory for Biology of Plant Diseases and Insect Pests, Institute of Plant Protection, Chinese Academy of Agricultural Sciences, Beijing China
2 School of Horticulture and Plant Protection, Yangzhou University, Yangzhou Jiangsu Province, China
Jing‐Fei Guo
Stuart r. reitz.
3 Malheur Experiment Station, Oregon State University, Ontario OR, USA
Zhong‐Ren Lei
Sheng‐yong wu, associated data.
Western flower thrip, Frankliniella occidentalis (Pergande), is among the most economically important agricultural pests globally, attacking a wide range of vegetable and horticultural crops. In addition to causing extensive crop damage, the species is notorious for vectoring destructive plant viruses, mainly belonging to the genera Orthotospovirus , Ilarvirus , Alphacarmovirus and Machlomovirus . Once infected by orthotospoviruses, thrips can remain virulent throughout their lifespan and continue transmitting viruses to host plants when and wherever they feed. These irruptive viral outbreaks in crops will permanently disrupt functional integrated pest management systems, and typically require a remedial treatment involving insecticides, contributing to further development of insecticide resistance. To mitigate against this continuing cycle, the most effective management is early and comprehensive surveillance of the pest species and recognition of plant viruses in the field. This review provides information on the pest status of F. occidentalis , discusses the current global status of the viruses vectored by this thrip species, examines the mechanisms involved in transmitting virus‐induced diseases by thrips, and reviews different management strategies, highlighting the potential management tactics developed for various cropping systems. The early surveillance and the utilization of potential methods for control of both F. occidentalis and viruses are proposed.
Introduction
Thrips (order Thysanoptera) are minute insects only a few millimeters or less in length. Of the approximately 5500 described species of thrips in the world, scarcely 1% are considered to be serious pests of commercial crops (Morse & Hoddle, 2006 ; Healey et al ., 2017 ). Among these pests, several species stand out as being among the most important global agricultural pests. These include four of the major thrip pests, the western flower thrip, Frankliniella occidentalis Pergande, the onion thrip, Thrips tabaci Lindeman, the melon thrip, T. palmi Karny and the yellow tea thrip (chili thrip), Scirtothrips dorsalis Hood (Mound, 2002 ; Riley et al ., 2018 ). F. occidentalis is a polyphagous and ubiquitous invader of key agri‐ and horticultural crops in diverse field and greenhouse environments. This is due to the damage caused directly by its feeding and oviposition, and indirectly through transmission of plant viruses, of which tomato spotted wilt orthotospovirus (TSWV) is the most economically important (Schneweis et al ., 2017 ). F. occidentalis was first described in 1895 in California, USA, and beginning in the late 1970s has since become a major global pest (Kirk & Terry, 2003 ). This species has been the most intensively studied member of the order Thysanoptera since 1980, accounting for over one‐third of the publications based on this order (Reitz et al ., 2011 ). F. occidentalis has continued its spread around the world and is now distributing in at least 57 countries (Fig. 1 , Table S1 ). The spread of F. occidentalis and various vectored orthotospoviruses have frequently caused failure of established integrated pest management (IPM) systems for agricultural crops (Morse & Hoddle, 2006 ).

The worldwide distribution of Frankliniella occidentalis . GS (2019) 4551.
Phytophagous thrips have many traits that predispose them to be successful invaders, such as minute size, cryptic habits, high reproductive potential and high dispersal capability. F. occidentalis has superior or additional features, promoting their worldwide spread and sustained damage. The possible reasons for the start of the spread is intensive insecticide use in the 1970s and 1980s, which was reviewed by Kirk and Terry ( 2003 ). Biological factors facilitating invasion by thrips was reviewed by Morse and Hoddle ( 2006 ). Biological processes and molecular interactions involved in the virus acquisition and transmission by thrips was reviewed by Whitfield et al . ( 2005a ). Over a decade after these reports, we aim to provide a summary of the extraordinary attributes that make for a successful invader with major economical damage potential. We have reconstructed in a chronological order the current global distribution of F. occidentalis , as well as several viruses transmitted. We have also summarized the control strategies based on IPM of F. occidentalis , stressing the recent progress in biological control.
Biology and ecology
F. occidentalis possesses several biological and ecological characteristics that enable it to become a dominant thrip species in many of the areas it has invaded. Its short generation time and high reproductive potential, often with a predisposition to parthenogenesis, enhances the likelihood of establishment (Kirk & Terry, 2003 ; Zhang et al ., 2010 ); its cryptic behavior and high level of vagility enable it to disperse to a wide variety of crops (Cloyd, 2009 ; Reitz, 2009 ); its polyphagous nature likely supplements its predisposition to evolve resistance to many classes of insecticides through metabolic detoxification pathways (Demirozer et al ., 2012 ); its widespread resistance to most major insecticides, in turn, makes it difficult to control (Bielza, 2010 ; Gao et al ., 2012a ); its highly efficient exploitation of food sources provides it with a competitive advantage over indigenous species and enables it to become successfully established in new regions (Morse & Hoddle, 2006 ; Demirozer et al ., 2012 ). However, its propensity to transmit viruses often results in serious losses in a wide range of crops (Wijkamp et al ., 2010 ; Ogada & Poehling, 2015 ). In most cases, combinations of these attributes contribute to its high invasion success, ultimately resulting in severe economic damage to crops throughout the world.
Viruses transmitted by F. occidentalis
Thrips are the only known vectors of orthotospoviruses, but only 0.16% of the known species have been implicated in their transmission (Mound, 2004 ). Thrips transmit viruses belonging to at least four virus groups, including ilarviruses, machlomoviruses, alphacarmoviruses and orthotospoviruses (Fig. 2 , Table S2 ). (Jones, 2005 ; Morse & Hoddle, 2006 ). Using TSWV as an example, this particular virus, which is one of the most economically important members of the genus Orthotospovirus ( Tospoviridae ) (Mumford et al ., 1996 ), has long been associated with F. occidentalis – one of the most important and efficient vector thrips (Wijkamp, 1995a ; Arthurs et al ., 2018a ). TSWV acquisition by F. occidentalis is a developmental‐stage dependent process, with the 1st instar larval stage considered as the most susceptible phase (Rotenberg et al ., 2015 ). The interactions between TSWV and F. occidentalis and its dissemination route in thrips has been thoroughly reviewed by Whitfield et al . (2015a), Rotenberg et al . ( 2015 ) and Dietzgen et al . (2016). TSWV is acquired by the thrips’ stylets and travels across the alimentary canal to the anterior region of the midgut (MG), where the surface glycoproteins, Gn and Gc, facilitate its entrance into the thrips’ MG (Whitfield et al ., 2004 , 2005b ). Subsequently, TSWV replicates and accumulates in the visceral muscles of the gut, later spreading back into the salivary glands through the connected ligaments, and is then transmitted to the plant by the stylets (Fig. 3 ). Abe et al . ( 2011 ) suggested that TSWV infection facilitates the production of thrips of the next generation, which will contribute to further spread of TSWV. Stafford et al . ( 2011 ) reported that TSWV infection directly influences the feeding behavior of thrips, and enhances the transmission efficiency of the virus, whereas, viruses such as ilarviruses are also thought to be transmitted very transiently by F. occidentalis (Aramburu et al ., 2010 ), where the transmission starts when the thrips feed on virus‐laden pollen and ends once the virus‐laden pollen is gone.

The worldwide distribution of viruses transmitted by Frankliniella occidentalis . The viruses shown in the map are: AlNSV, alstroemeria necrotic streak orthotospovirus; CSNV, chrysanthemum stem necrosis orthotospovirus; GRSV, groundnut ringspot orthotospovirus; INSV, impatiens necrotic spot orthotospovirus; TCSV, tomato chlorotic spot orthotospovirus; TSWV, tomato spotted wilt orthotospovirus; TYRV, tomato yellow ring virus; TZSV, tomato zonate spot orthotospovirus; PMoV, parietaria mottle virus; PFBV, pelargonium flower break virus; MCMV, maize chlorotic mottle virus. Arcgis 10.0 software was used to create the map. GS (2019) 4551.

Virus localization sites in Frankliniella occidentalis . Viruses are initially acquired through the stylets. They then travel across the alimentary canal to the anterior region of the midgut (MG), where the surface glycoproteins, Gn and Gc, facilitate their entrance into the thrips’ MG. Subsequently, the viruses replicate, accumulate in the visceral muscles of the gut, and then spread to the salivary glands through the connective ligaments, where they are then transmitted back to the plants through the stylets.
Current global status of the viruses transmitted by F. occidentalis
Presently, a total of 11 viruses have been reported vectored by F. occidentalis . These include eight species in the genus Orthotospovirus ( Tospoviridae ): alstroemeria necrotic streak orthotospovirus (AlNSV), chrysanthemum stem necrosis orthotospovirus (CSNV), groundnut ringspot orthotospovirus (GRSV), impatiens necrotic spot orthotospovirus (INSV), tomato chlorotic spot orthotospovirus (TCSV), TSWV, tomato yellow ring virus (TYRV), and tomato zonate spot orthotospovirus (TZSV); parietaria mottle virus (PMoV) in the genus Ilarvirus ( Bromoviridae ); and pelargonium flower break virus (PFBV) in the genus Alphacarmovirus , and maize chlorotic mottle virus (MCMV) in the genus Machlomovirus (both in Tombusviridae ) (Fig. 2 , Table S2 ). The global distributions, hosts, emergence and dissemination of these viruses are discussed below.
AlNSV was first described in Colombia, when it was found to cause necrotic streaks on the leaves of Peruvian lilies ( Alstroemeria sp.) (Hassani‐Mehraban et al ., 2010 ). According to the nucleocapsid (N) protein gene sequence, phylogenetic analysis revealed that AlNSV clustered with those orthotospoviruses from the American continent into a single lineage with a significantly close serological relationship (Hassani‐Mehraban et al ., 2010 ; Liu et al ., 2017 ). Similar to other reference orthotospoviruses, AlNSV is capable of infecting ornamentals as well as vegetables locally or systemically, and is transmitted by F. occidentalis under experimental conditions (Hassani‐Mehraban et al ., 2010 ).
CSNV was first identified from chrysanthemums in Brazil in 1996 (Resende et al ., 1996 ), followed by the Netherlands, Slovenia, UK, Japan and South Korea (Resende et al ., 1996 ; Verhoeven et al ., 1996 ; Mumford et al ., 2003 ; Ravnikar et al ., 2003 ; Okuda et al ., 2013 ; Yoon et al ., 2017a ). In Brazil, CSNV also infects tomatoes with necrosis and necrotic spots on the stem and leaves, showing symptoms similar to those seen in chrysanthemums (Bezerra et al ., 1999 ; Nagata et al ., 2004 ). F. schultzei and F. intonsa have also been identified as vectors of CSNV although their efficiencies as vectors are much lower than F. occidentalis (Nagata et al ., 2004 ; Okuda et al ., 2013 ).
GRSV was first described from South Africa and Brazil from peanuts and tomatoes, respectively (Wijkamp, 1995b ; Pappu et al ., 2009 ). It was subsequently reported from Argentina, the USA, the Caribbean basin and Ghana from a relatively narrow host range compared to TSWV (Webster et al ., 2010 ; Webster et al ., 2011 ; Camelo‐Garca et al ., 2014 ; Spadotti et al ., 2014 ; Leão et al ., 2015 ; Webster et al ., 2015 ; Appiah et al ., 2016 ). In Brazil, GRSV was identified from several hosts including sweet peppers, coriander, cocona, cucumbers, cubiu, peanuts and watermelons (Lima et al ., 1999 ; Boari et al ., 2002 ; Camelo‐Garca et al ., 2014 ; Spadotti et al ., 2014 ; Leão et al ., 2015 ). In North America, GRSV was initially reported from tomatoes in south Florida in 2009, subsequently from peppers, tomatilloes and eggplants in peninsular Florida, and later in South Carolina and New York (Webster et al ., 2010 , 2011 , 2015 ). Interestingly, a reassortant isolate GRSV‐L G M T S G , composed of the L and S RNAs from GRSV and the M RNA from TCSV, was reported from tomatoes in Florida in 2010 (Webster et al ., 2011 ). The recent outbreaks of GRSV in Brazil and North America were probably driven by its major thrip vectors, F. occidentalis , F. schultzei , and F. gemina (Pappu et al ., 2009 ; Gilbertson et al ., 2015 ; Webster et al ., 2015 ). Among the three species involved, F. schultzei , the local species from Brazil and North America, has a more efficient transmission than the other two thrip species (Nagata et al ., 2004 ; Gilbertson et al ., 2015 ; Webster et al ., 2015 ).
INSV, which is considered to be an important pathogen of ornamental crops, was initially characterized and distinguished from New Guinea impatiens in the Netherlands in the late 1980s (Ávila et al ., 1992 ). It is now widespread throughout much of the world (Vaira et al ., 1993 ; Peters et al ., 1996 ; Lebas & Ochoa‐Corona, 2007 ; Pappu et al ., 2009 ). In northern Africa, the Middle East, Southeast Asia, southern New Zealand, the Caribbean and Central America, INSV has been reported from numerous field and greenhouse‐grown ornamentals, including freesia, impatiens, lobelia, primula, ranunculus, begonia, chrysanthemum, and so on, (Lebas et al ., 2004 ; Jones, 2005 ; Lebas & Ochoa‐Corona, 2007 ; Pappu et al ., 2009 ), as well as a number of weed species (Okuda et al ., 2010 ). Traditionally, INSV was also believed to be a pathogen on some vegetable crops, although it is only capable of causing limited local symptoms or is symptomless on sweet peppers, pepinos, spinach, tomatoes and cucumbers (Verhoeven & Roenhorst, 1998 ; Vicchi et al ., 1999 ; Sialer & Gallitelli, 2000 ; Mavric & Ravnikar, 2001 ). However, INSV has recently emerged as an important pathogen of lettuce caused by F. occidentalis transferring from ornamental hosts in coastal regions of California (Pappu et al ., 2009 ; Kuo et al ., 2014 ; Gilbertson et al ., 2015 ). In addition to F. occidentalis , INSV can also be transmitted by F. intonsa and F. fusca , but with a lower efficiency (Naidu et al ., 2001 ; Sakurai et al ., 2004 ).
In 1990, TCSV was first characterized as a distinct serotype of TSWV from tomatoes in Brazil (De Avila et al ., 1990 , 1993 ). Subsequently, TCSV was isolated from sweet peppers, potatoes, endives, celery, lisianthus and various weeds with mosaic, necrosis, chlorotic or stunting symptoms in Argentina and Brazil (Boiteux et al ., 1993 ; Gracia et al ., 1999 ; Colariccio et al ., 2001a ; Dal Bio et al ., 2001 ; Eiras et al ., 2002 ), and from outbreaks on lettuce and gilo in Brazil (Colariccio et al ., 2001b ; Rabelo et al ., 2002 ). In the USA, TCSV was first detected from tomatoes in south Florida in 2012 (Londoño et al ., 2012 ), and then from lettuce, impatiens and peppers (Webster et al ., 2015 ). In Puerto Rico, TCSV was also found from tomatoes, peppers, jimsonweed ( Datura stramonium ), and lettuce in 2013 (Estévez de Jensen et al ., 2013 ; Estévez de Jensen & Adkins, 2014 ). More recently, TCSV was identified from tomatoes in the Dominican Republic (Batuman et al ., 2014 ).
At the beginning of the 20th century, spotted wilt disease of tomato was first described in Australia in 1915 (Brittlebank, 1919 ). Afterwards, it was considered as a viral disease caused by TSWV and transmitted by T. tabaci and F. schultzei (Pittman, 1927 ; Samue et al ., 1930 ). Normally, TSWV‐infecting tomatoes shows bronzing, curling necrotic streaks and spots on the leaves, and a paler red or yellow skin color on the fruits. In addition to tomatoes, TSWV was subsequently isolated from many other plants such as aubergine, artichoke, bell peppers, cabbage, chrysanthemums, cowpeas, cucumbers, butternut squash, hot peppers, common beans, lettuce, petunias, papaya, peas, peanut, eggplants, pineapples, potatoes, strawberries, mangos, soybeans, celery, spinach, sweet peppers, broad beans, tobacco, cauliflower and assorted weeds (Chatzivassiliou et al ., 2000a ; Jones, 2005 ; Whitfield et al ., 2005a ; Reitz et al ., 2011 ).
In Europe, TSWV was first described in England in 1929 from ornamental winter cherries ( Solanum capsicastrum ) with concentric ring symptoms on leaves (Smith, 1932 ). In the early 20th century, TSWV was transmitted mainly by T. tabaci . An obvious decline of TSWV‐infecting crops was evident when effective controls successfully managed this vector. When F. occidentalis extended its range to Europe, TSWV began to be a major threat to European horticultural crops (Jones, 2005 ). Today, TSWV is well established in almost all European countries, including Albania (Cota & Merkuri, 2004 ), Bulgaria (Dikova et al ., 2013 ), Bosnia and Herzegovina (Trkulja et al ., 2013 ), France (Marchoux et al ., 1991 ), Greece (Chatzivassilou et al ., 1996 ; Chatzivassiliou et al ., 2000b ), Hungary (Salamon et al ., 2012 ), Montenegro (Zindović et al ., 2011 ), Spain (Jorda, 1993 ; Aramburu et al ., 1997 ), Portugal (Louro, 1996 ), and Slovenia (Mavric & Ravnikar, 2001 ). In Serbia, TSWV was isolated from Gerbera hybrida in 2009 (Stanković et al ., 2011 ), onions, garlic and chrysanthemums in 2011 (Stanković et al ., 2012 , 2013 ), and Brugmansia sp. in 2012 (Nikolić et al ., 2013 ).
In Africa, TSWV was first described from a wilt disease of tobacco in South Africa as early as 1905 (Moore, 1933 ), and later found in several provinces of the country infecting tobacco, tomatoes, peppers and potato crops (Moore & Andessen, 1939 ). After F. occidentalis was introduced into Africa, TSWV became widespread in other African countries (Moussa et al ., 2000 ; Ben Moussa et al ., 2005 ). Recently, TSWV has been found from Amaranthus thunbergii in South Africa (Kisten et al ., 2016 ), butternut squash ( Cucurbita moschata ) and peppers in Zimbabwe in 2015 (Karavina et al ., 2016a , b ).
In Asia, TSWV was first recorded in the Middle Eastern countries. In July 1998, TSWV was identified from Pittosporum tobira shrubs with foliar ring spots, mild mosaic, and tip necrosis symptoms in a nursery in the Sharon Valley of Israel (Gera et al ., 2000a ), and later from several vegetables (Gera et al ., 2000b ). Similarly, the virus was also detected from potatoes in 1998 in Iran (Pourrahim et al ., 2001 ), and subsequently from soybeans, tomatoes, and cucurbits (Golnaraghi et al ., 2001 ; Massumi et al ., 2007 , 2009 ). TSWV has also been isolated from many important vegetable crops in Jordan and Lebanon (Anfoka et al ., 2006 ; Abou‐Jawdah et al ., 2006 ). More recently, TSWV was reported from lettuce showing necrotic lesions, necrosis of the lamina of the younger leaves, and leaf curling symptoms in March 2014 from the Al‐Uyaynah area, in the central region of Saudi Arabia. In eastern Asia, TSWV is now widely distributed in China, Korea, and Japan in a number of vegetable and horticultural crops such as celery, peppers, cowpeas, lettuce, Bidens pilosa , tomatoes, potatoes, Brugmansia suaveolens , Eustoma grandiflorum , and miscellaneous other wild plant species (Choi et al ., 2004 ; Zheng et al ., 2010 ; Reitz et al ., 2011 ; Okazaki et al ., 2007 , 2011 ; Choi & Choi, 2015 ; Li et al ., 2015 ; Xiao et al ., 2016 ; Yoon et al ., 2017b ). In India, TSWV was found on sunflowers exhibiting severe mosaic, systemic necrosis, leaf distortion, and ringspots symptoms in Tirupati in January 1998 (Subbaiah et al ., 2000 ), and more recently from chrysanthemums grown in the Nilgiris district of Tamil Nadu State in August 2013 (Renukadevi et al ., 2015 ).
In America and the Caribbean, TSWV was first discovered from pineapples causing yellow spot disease as early as 1926 in Hawaii (Kucharek et al ., 2000 ). In the 1970s, the virus was reported from peanuts in Texas (Haliwell & Philley, 1974 ), and then from peppers, tobacco and tomatoes in Georgia and other areas of the southeastern USA in the mid‐1990s (Culbreath et al ., 1991 ). In the latest 20 years, TSWV has become widespread throughout most of the states in the USA (Groves et al ., 1998 ; Holcomb et al ., 1999 ; Díaz‐Pérez & Pappu, 2000 ; Holcomb & Valverde, 2000 ; Momol et al ., 2000 ; Adkins et al ., 2003 ; Whitfield et al ., 2003 ; Mullis et al ., 2004 ; Yang et al ., 2004 ; Adkins & Baker, 2005 ; Mullis et al ., 2006 ; Nischwitz et al ., 2006a , b ; Baker et al ., 2007 ; Baker et al ., 2009 ; Barkley et al ., 2009 ; Crosslin et al ., 2009 ) causing significant economic losses (Pearce, 2005 ). More recently, TSWV was isolated from Stevia rebaudiana and tomatoes with the Sw‐5 orthotospovirus‐resistance gene in Carolina (Koehler et al ., 2016 ; Batuman et al ., 2017 ). TSWV was found in a commercial chrysanthemum field of Mexico infesting several weeds including Taraxacum officinale , Bidens sp., Reseda luteola , Mirabilis jalapa being transmitted by F. occidentalis (Martinez et al ., 1999 ). In 2005 to 2006, tomatoes showing chlorosis, malformation of apical leaves, stunting, and ringspot lesions caused by TSWV were first noticed in the Baja California peninsula of Mexico (Holguín‐Peña & Rueda‐Puente, 2007 ). In the Dominican Republic, TSWV transmitted by F. occidentalis was found to be widely distributed in commercial peppers and tomatoes growing under protected greenhouse conditions (Martínez et al ., 2014 ). In South America, tomato, pepper, and lettuce crops infected by TSWV were reported from Argentina, Brazil, Chile, and Venezuela causing a significant threat to the vegetable industry (Maluf et al ., 1991 ; Gracia et al ., 1999 ; EPPO, 2004 ; Lebas & Ochoa‐Corona, 2007 ; Rosales et al ., 2007 ; Marys et al ., 2014 ; Pérez‐Colmenares et al ., 2015 ).
The first recognition that tomato spotted wilt disease was caused by TSWV occurred in Australia as early as 1915, although it was considered as an introduction from elsewhere following European colonization (Brittlebank, 1919 ; Pittman, 1927 ; Samuel et al ., 1930 ; Smith, 1932 ). In Australia, TSWV was mainly transmitted by T. tabaci and F. shultzei on several vegetables with limited spread occurring over a span of many decades. When F. occidentalis was detected in southwestern Australia in 1993, an outbreak of TSWV was being reported in eastern and southeastern Australia (Latham & Jones, 1997 ; Wilson et al ., 2000 ; Pappu et al ., 2009 ). Similarly, TSWV was detected from tomatoes and other vegetables in New Zealand soon after its discovery on the Australian continent, and, at the time, was also transmitted by T. tabaci (Chamberlain & Taylor, 1936 , 1938 ). More recently, a serious epidemic of TSWV on ornamental plants grown in greenhouses on the North Island was caused by F. occidentalis rather than T. tabaci (Fletcher et al ., 2005 ; Pappu et al ., 2009 ).
TYRV, a tentative orthotospovirus species, is closely related to iris yellow spot virus. The virus was first identified from tomatoes in Teheran Province, Iran (Hassani‐Mehraban et al ., 2005 ). Subsequently, TYRV was isolated from chrysanthemums, gazanias, potatoes, soybeans, and cineraria with high diversity in the N gene in Iran (Hassani‐Mehraban et al ., 2005 , 2007 ; Rasoulpour & Izadpanah, 2007 ). In 2012, TYRV was isolated from tomatoes with chlorotic ring spots on fruits and necrosis of stems and leaves in Kenya (Birithia et al ., 2012 ). TYRV has now been found in Europe in tomato plants having symptoms of necrosis on leaves and stalks, and chlorotic and necrotic ringspots on fruits in Kujawsko‐Pomorskie Province, Poland (Zarzyńska‐Nowak et al ., 2016 ).
TZSV was first reported to naturally infect tomatoes, causing zoned ring spots on fruits in Yunnan Province, China (Dong et al ., 2008 ). In Yunnan Province, TZSV was subsequently isolated from chili peppers ( Capsicum annuum ), peppers, tobacco, Iris tectorum , potatoes and several weeds, including Bidens pilosa and Rumex dentatus (Dong et al ., 2010 ; Zheng et al ., 2014 ; Huang et al ., 2015 ; Liu et al ., 2015 ; Wu et al ., 2016a ). During a survey from 2008 to 2010 in Guangxi Province, China, TZSV was also detected from tobacco. Infection symptoms included dwarfing, midrib browning, distorted apical buds, and concentric ringspot (Cai et al ., 2011 ).
PMoV, a member of the genus Ilarvirus , was originally isolated from the weed Parietaria officinalis in 1989 (Caciagli et al ., 1989 ), and afterwards from tomato, Mirabilis jalapa , Capsicum annuum , Diplotaxis tenuifolia in Italy (Roggero et al ., 2000 ; Parrella, 2002 ; Parrella et al ., 2016 , 2017 ). Besides Italy, PMoV has also been detected from tomatoes in France, Spain and Greece, and from Capsicum annuum in Spain (Ramasso et al ., 1997 ; Roggero et al ., 2000 ; Aramburu, 2001 ; Galipienso et al ., 2005 ; Janssen et al ., 2005 ).
PFBV is a member of the genus Alphacarmovirus , affecting Pelargonium spp. which causes white flower streaking, chlorotic spotting of leaves and stunting on some cultivars. It was originally identified in Europe and has now spread throughout much of the world (Stone & Hollings, 1973 ; Bouwen & Maat, 1992 ; Blystad et al ., 1995 ; Ivars et al ., 2004; Rico et al ., 2004 ; Rico & Hernández, 2006 ; Rico et al ., 2006 ; Wei et al ., 2015 ). It is primarily known for its detrimental effects on the production and quality of some Pelargonium spp. cultivars (Bouwen & Maat, 1992 ; Blystad et al ., 1995 ; Krczal et al ., 1995 ; Ivars et al ., 2004; Wei et al ., 2015 ). PFBV is frequently transmitted and dispersed by vegetative propagation and irrigation systems as well as by the western flower thrip, F. occidentalis (Krczal et al ., 1995 ).
MCMV, a member the genus Machlomovirus in the family Tombusviridae , was first identified from maize in the Americas including plants from Peru and the USA (Castillo‐Loayza, 1977 ; Niblett & Clafin, 1978 ; Jiang et al ., 1990 ). In maize, MCMV is among the important pathogens that characteristically induce typical symptoms in the plants such as mosaicism, stunting and necrosis (Niblett & Clafin, 1978 ; Mahuku et al ., 2015 ; Chen et al ., 2017 ). MCMV, together with other maize‐infecting potyviruses, are responsible for inducing corn lethal necrosis disease, which was first described in Peru in 1974 and has since spread worldwide (Castillo‐Loayza, 1977 ; Niblett & Clafin, 1978 ; Morales et al ., 1999 ; Adams et al ., 2014 ; Deng et al ., 2014 ; Lukanda et al ., 2014 ; Gowda et al ., 2015 ; Mahuku et al ., 2015 ; Quito‐Avila et al ., 2016 ; Chen et al ., 2017 ). In addition to maize, MCMV also can infect sorghum, Coix seed and finger millet in several Asia and Africa regions, probably due to its diverse transmission methods including by seeds, mechanical inoculation, and insects including thrips and beetles (Jiang et al ., 1990 ; Cabanas et al ., 2013 ; Deng et al ., 2014 ; Kusia et al ., 2015 ; Achon et al ., 2017 ; Chen et al ., 2017 ).
In conclusion, from the emergence and dissemination of F. occidentalis and its transmitting viruses, we speculate that the western flower thrip has spread from its original distribution in western North America to tropical, subtropical, temperate and cold temperate zones of the world by the movement of horticultural material, such as cuttings, seedlings and potted plants, while the spread of F. occidentalis‐ transmitting viruses is shared along with the migration pattern (or trends) of its vector, especially TSWV and INSV. F. occidentalis was rarely reported in cold zones especially in areas with temperature dropping to −10 °C in winter which is 100% lethal to F. occidentalis attempting to overwinter outdoors.
Management of F. occidentalis
Because of their small size and the difficulty involved in detection and identification, successful invasions of thrips often occur unnoticed. As a result, F. occidentalis has become a major global pest with immense damage potential in only 30 years. In addition, adults are capable of migrating long distances to new host plants and are able to quickly transmit their viruses (Kliot et al ., 2016 ). With these risks in mind, the most effective means of dealing with this potentially invasive and pestiferous thrip species is to prevent its entry and establishment into nonendemic regions. For example, methyl bromide was used for post‐harvest fumigation of a number of commodities either by the exporting or importing country (provinces) after a thrip infestation is noticed (Morse & Hoddle, 2006 ). However, because of its ozone depletion effect, methyl bromide is being phased out worldwide (Deewatthanawong et al ., 2016 ). The alternatives to methyl bromide include irradiation, sulfuryl fluoride, phosphine, ethanedinitrile, low oxygen treatments, heat and cold treatments (Cox, 2017 ). A number of sustainable tactics have been developed in IPM programs for managing F. occidentalis and to inhibit its persistent spread worldwide with ever increasing damage to its many host crops.
Chemical control
Management of F. occidentalis has been a difficult task. Use of insecticides has traditionally been the primary strategy for control of F. occidentalis , especially in virus‐sensitive crops (Bielza, 2008). The insecticides that are normally applied can be separated into two major groups: broad‐spectrum insecticides, which include pyrethroids, neonicitinoids, organophosphates and carbamates, and narrow‐spectrum insecticides, which include pyridalyl and lufenuron (Mouden et al ., 2017 ). However, frequent applications of insecticides, especially those containing pyrethroids, organophosphates, neonicitinoids and carbamates have also decimated large percentages of natural enemies and led to the rapid development of insecticide resistance in F. occidentalis (Mouden et al ., 2017 ). This propensity of F. occidentalis for developing insecticide resistance has been a primary factor in promoting its pest status.
Spinosad and the related spinetoram, which tend to be compatible with natural enemies, are now being extensively used and currently provide the most effective chemical control of F. occidentalis (Gao et al ., 2012a ; Li et al ., 2016 ). However, the applications of any insecticide will eventually contribute to resistance development in a given pest species. Evidence has shown that Spinosad resistance is now present in some populations of F. occidentalis in the USA (Weiss et al ., 2009 ), Australia (Herron et al ., 2014 ) and China (Li et al ., 2016 ). If deemed necessary, insecticide use should be accurate, precise and complement other compatible control approaches.
Agricultural practices
Thrips often overwinter in patches of uncultivated plants and migrate into cropping systems in the spring (Pearsall & Myers, 2000 ), with cropping systems often serving as a sink, with sources of insect populations occurring in field margins and fencerows. F. occidentalis , which is a highly polyphagous pest of many cultivated as well as wild plants, has been shown to feed on more than 240 host plants (Tommasini & Maini, 1995 ), including many weed species. In France, the weed, gallant soldier ( Galinsoga parviflora Cav.), has been reported by Nyasani et al . ( 2013 ) as an excellent host of F. occidentalis for both feeding and reproduction under field conditions, and serves as a potential source of thrip outbreaks in French bean fields. In instances such as this, where alternative hosts are identified and known to be present, sound agricultural practices such as seasonal mowing of these weeds will likely decrease the number of thrips migrating into the cropping systems (Northfield et al ., 2008 ).
Additional practices for managing F. occidentalis include creating a less favorable environment by irrigation to reduce numbers of F. occidentalis adults (Schuch et al ., 1998 ), by decreasing the levels of nitrogen fertilization to reduce populations of F. occidentalis in ornamentals (Brodbeck et al ., 2001 ; Chow et al ., 2012 ), and by growing trap plants to draw F. occidentalis away from susceptible crops, thereby reducing the number of thrips on the target crop (Cook et al ., 2006 ).
Physical control
Because of their small size, fine mesh screens have been widely used to cover greenhouse openings such as vents to help physically prevent thrips from immigrating onto protected crops (Arthurs et al ., 2018b ). It was reported by Tinoco et al . ( 2014 ) that using appropriate mesh size screens on greenhouse windows would reduce the incidence of F. occidentalis by 20% in protected tomatoes. Because thrips find suitable host plants by utilizing different cues, including visual cues in the ultraviolet (UV) spectrum (Terry, 1997 ), using materials that reflect UV radiation can obscure their host‐locating cues. Several researchers have found that using UV‐reflective mulch significantly reduced early season abundance of adult thrips and disease incidence (Stavisky et al ., 2002 ; Kigathi & Poehling, 2012 ).
Another conventional measure, sticky cards, are widely used by growers for monitoring thrip populations in greenhouses (Ren et al ., 2008 ). It was reported that blue cards are highly attractive to F. occidentalis (Otieno et al ., 2018 ). Because adult thrips explore their host range in part through volatiles, the commercially available F. occidentalis semiochemicals are frequently used as lures in conjunction with sticky card traps (Broughton et al ., 2015 ) to attract and monitor or eliminate thrips.
Biological control
There has been considerable interest in the use of biological control agents to reduce thrip populations, especially in protected crops. An effective use of agents has been shown to improve thrip management. Inoculative release of agents, beginning at crop initiation before the resident thrips approach economically damaging levels, is recommended (Reitz et al ., 2011 ). The large number of biological control agents that have been reported to attack F. occidentalis can be separated into two groups: macrobials (predators and parasitoids) and microbials (fungal pathogens and entomopathogenic nematodes) (Mouden et al ., 2017 ). The macrobials currently being widely and effectively used are anthocorid bugs ( Orius spp.) (Mo et al ., 2013 ; Aragón‐Sánchez et al ., 2018 ), green lacewing species (Sarkar et al ., 2019 ) and predatory phytoseiid mites (Messelink et al ., 2006 ; Ahmed & Lou, 2018 ), which predominantly attack 1st instar thrips on foliage, and soil‐dwelling predaceous laelapid mites (Berndt et al ., 2004 ; Wu et al ., 2016b ), which consume thrip pupae in soil.
Fungal pathogens used as biocontrol agents of F. occidentalis , are Beauveria bassiana (Gao et al ., 2012b ; Lee et al ., 2017 ), Metarhizium anisopliae (Maniania et al ., 2003 ; Toledo‐Hernández et al ., 2017 ) and Lecanicillium lecanii (Gouli et al ., 2009 ; Wang et al ., 2013 ). The various nematode species used against soil‐inhabiting pupae of F. occidentalis are in the genera Steinernema and Heterorhabditis (Ebssa et al ., 2004 ; Buitenhuis & Shipp, 2005 ). There is currently interest in combinations of different biological control agents, which may result in an additive suppression of F. occidentalis populations (Messelink & Janssen, 2014 ; Saito & Brownbridge, 2016 ; Wu et al ., 2017 ). Furthermore, fungal‐based granular formulations of entomopathogenic fungi are regarded as an effective strategy for thrip management by controlling the soil‐dwelling developmental stages of thrips (Lee et al ., 2017 ). The latest research demonstrates that the application of B. bassiana granules to the soil surface can successfully suppress F. occidentalis under greenhouse conditions (Zhang et al ., 2019 ).
Concluding remarks
With the continuing increase in global trade in ornamental greenhouse plants, it is likely that F. occidentalis will continue its rapid spread into, as yet, uninfested areas around the world, causing substantial amounts of damage from feeding and virus transmission. Another consideration is that F. occidentalis may also be capable of expanding its range to new areas as a consequence of global climate warming (Wu et al ., 2018 ). Considering the economic importance of F. occidentalis both as a pest and a vector of several notorious plant viruses, it is essential to establish early surveillance systems of the species and to encourage the rapid recognition of plant virus symptoms while keeping a constant vigil on further spread of the species, especially in cold zones where F. occidentalis has not been reported.
Aggregation pheromones of F. occidentalis have been identified and shown to be cost‐effective for monitoring detection of this thrip species in the field (Kirk, 2017 ). Huang et al . ( 2010 ) and Zhang et al . ( 2012 ) provided a diagnostic polymerase chain reaction detection system, which can quickly and accurately identify F. occidentalis from thrip larvae to complement the traditional morphological identification. This method can also be used for on‐site testing of samples at ports‐of‐entry in the future. Standard area diagrams (SADs) have been used as a tool to improve the accuracy and reliability of visual estimates of leaf spotting diseases. More than 100 diseases with a range of plant organs were validated by SAD (Del Ponte et al ., 2017 ). Presently, enhanced accessibility of cameras and image analysis software has accelerated the development of more realistic, stylized color representations or diagrams based on photographs of diseased plant organs (Del Ponte et al ., 2019 ). Hence, we consider that it is a potential method for rapid recognition of F. occidentalis ‐transmitting virus symptoms in the near future.
Most of the vector thrip species have high fecundity, short reproductive cycles and extensive plant host ranges (Whitfield et al ., 2005a ). F. occidentalis populations tend to be efficient vectors of multiple orthotospovirus species. Although the research on biological processes involved in the transmission of orthotospoviruses and their thrip vectors has made progress during the last decade (Hogenhout et al ., 2008 ; Rotenberg et al ., 2015 ), there is still a lack of effective measures for management of F. occidentalis and its transmitted viruses. At present, there is still a heavy reliance on insecticides, which will continue to play an important role in thrip management in the foreseeable future. The increased incidence of F. occidentalis throughout the world that we are currently witnessing, could be a consequence of increased insecticide applications over the past 30 years. There is mounting evidence that synthetic pyrethroids can stimulate reproduction of F. occidentalis (Funderburk, 2009 ) and promote insecticide resistance (Gao et al ., 2012a ). Further studies, including the virus‐vector relationship of F. occidentalis with insecticide resistance are needed to improve our understanding of basic biological concepts and develop alternative measures for thrip control.
Although significant research progress in control of F. occidentalis has been made after using alternative measures, this thrip species continues to threaten the production of many crops worldwide because of the severity of viruses and difficulty in preventing thrip transmission. Therefore, both the thrips and plant virus diseases transmitted should be taken into account in control tactics. First, because immature F. occidentalis can be found consistently in tomato blossoms (Beaudoin, 2011 ) and have been shown to acquire TSWV from infected tomato and then transmit to susceptible plants (Szostek et al ., 2017 ), it was suggested that management of F. occidentalis infestations during the blooming season may be important for effective control of TSWV in susceptible tomato cultivars (Houle & Kennedy, 2017 ). Second, because adult thrips oviposit in plant tissue and prefer tight spaces, contact insecticides are often not effective against thrips. Induced systemic resistance (ISR) has recently gained more interest and might be the important option for management of thrips and transmitted virus (Mouden et al ., 2017 ). It was reported that Pseudomonas strains induced resistance against virus diseases (Vasanthi et al ., 2010 ); the combination of ISR by Pseudomonas and Neem oil, would be a best alternative in the future (Vasanthi et al ., 2017 ). In addition, integration of new biotechnology‐based strategies, as well as advances in computational systems will provide a powerful tool to drive innovation in reducing virus transmission and vector populations.
The authors declare no competing interests.
Supporting information
Table S1 . The worldwide distribution of Frankliniella occidentalis .
Table S2 . The worldwide distribution and host of viruses transmitted by Frankliniella occidentalis .
Acknowledgments
We wish to thank Dr. Cecil L. Smith (University of Georgia, USA) for help with the language editing of the manuscript. This work was supported by National Key Research & Developments (R&D) plan (Grant No. 2016YFD0201002), Key project at central government level: The ability establishment of sustainable use for valuable Chinese medicine resources (2060302) and the National Natural Science Foundation of China (No. 31601604).
The copyright for this article was changed on May 21 after original online publication.
- Abe, H. , Tomitaka, Y. , Shimoda, T. , Seo, S. , Sakurai, T. , Kugimiya, S . et al (2011) Antagonistic plant defense system regulated by phytohormones assists interactions among vector insect, thrips and a tospovirus . Plant Cell Physiology , 53 ( 1 ), 204–212. [ PubMed ] [ Google Scholar ]
- Abou‐Jawdah, Y. , El Mohtar, C. , Sobh, H. and Nakhla, M.K. (2006) First report of Tomato spotted wilt virus on tomatoes in Lebanon . Plant Disease , 90 , 376. [ PubMed ] [ Google Scholar ]
- Achon, M.A. , Serrano, L. , Clemente‐Orta, G. and Sossai, S. (2017) First report of Maize chlorotic mottle virus on a perennial host, Sorghum halepense , and maize in Spain . Plant Disease , 101 , 393. [ Google Scholar ]
- Adams, I.P. , Harju, V. , Hodges, T. , Hany, U. , Skelton, A. , Rai, S . et al (2014) First report of maize lethal necrosis disease in Rwanda . New Disease Reports , 29 , 22. [ Google Scholar ]
- Adkins, S. and Baker, C.A. (2005) Tomato spotted wilt virus identified in desert rose in Florida . Plant Disease , 89 , 526. [ PubMed ] [ Google Scholar ]
- Adkins, S. , Breman, L. , Baker, C.A. and Wilson, S. (2003) First report of Tomato spotted wilt virus in blackberry lily in North America . Plant Disease , 87 , 102. [ PubMed ] [ Google Scholar ]
- Ahmed, N. and Lou, M. (2018) Efficacy of two predatory phytoseiid mites in controlling the western flower thrips, Frankliniella occidentalis (Pergande) (Thysanoptera: Thripidae) on cherry tomato grown in a hydroponic system . Egyptian Journal Biological Pest Control , 28 , 15. [ Google Scholar ]
- Anfoka, G.H. , Abhary, M. and Stevens, M.R. (2006) Occurrence of tomato spotted wilt virus (TSWV) in Jordan . EPPO Bulletin , 36 , 517–522. [ Google Scholar ]
- Appiah, A.S. , Offei, S.K. , Tegg, R.S. and Wilson, C.R. (2016) Varietal response to groundnut rosette disease and thefirst report of groundnut ringspot virus in Ghana . Plant Disease , 100 , 946–952. [ PubMed ] [ Google Scholar ]
- Aragón‐Sánchez, M. , Román‐Fernández, L.R. , Martínez‐García, H. , Aragón‐García, A. , Pérez‐Moreno, I. and Marco‐Mancebón, V.S. (2018) Rate of consumption, biological parameters, and population growth capacity of Orius laevigatus fed on Spodoptera exigua . BioControl , 63 , 785–794. [ Google Scholar ]
- Aramburu, J. (2001) First report of Parietaria mottle virus on tomato in Spain . Plant Disease , 85 , 1210. [ PubMed ] [ Google Scholar ]
- Aramburu, J. , Galipienso, L. , Aparicio, F. , Soler, S. and López, C. (2010) Mode of transmission of Parietaria mottle virus . Journal of Plant Pathology , 92 , 679–684. [ Google Scholar ]
- Aramburu, J. , Laviña, A. , Moriones, E. , Riudavets, J. and Arnó, J. (1997) The proportion of viruliferous individuals in field populations of Frankliniella occidentalis : Implications for tomato spotted wilt virus epidemics in tomato . European Journal of Plant Pathology , 103 , 623–629. [ Google Scholar ]
- Arthurs, S.P. , Heinz, K.M. and Mitchell, F.L. (2018a) Comparison of Frankliniella fusca and Frankliniella occidentalis (Thysanoptera: Thripidae) as vectors for a peanut strain of tomato spotted wilt Orthotospovirus . Environmental Entomology , 47 , 623–628. [ PubMed ] [ Google Scholar ]
- Arthurs, S.P. , Krauter, P.C. , Gilder, K. and Heinz, K.M. (2018b) Evaluation of deltamethrin‐impregnated nets as a protective barrier against Western flower thrips, Frankliniella occidentalis (Thysanoptera: Thripidae) under laboratory and greenhouse conditions . Crop Protection , 112 , 227–231. [ Google Scholar ]
- Ávila, A.C. , Haan, P. De , Kitajima, E.W. , Kormelink, R. , Resende, R.D.O. , Goldbach, R.W . et al (1992) Characterization of a distinct isolate of Tomato spotted wilt virus (TSWV) from Impatiens sp. in the Netherlands . Journal of Phytopathology , 134 , 133–151. [ Google Scholar ]
- Baker, C.A. , Davison, D. and Jones, L. (2007) Impatiens necrotic spot virus and Tomato spotted wilt virus diagnosed in Phalaenopsis orchids from two Florida nurseries . Plant Disease , 91 , 1515. [ PubMed ] [ Google Scholar ]
- Baker, C.A. , Jones, L. , Leahy, R.M. and Soltis, D.E. (2009) Tomato spotted wilt virus found in five species of the genus Tragopogon in a Florida greenhouse . Plant Disease , 93 , 546. [ PubMed ] [ Google Scholar ]
- Barkley, N.A. , Pinnow, D.L. , Wang, M.L. and Pederson, G.A. (2009) First report of Tomato spotted wilt virus infecting African clover ( Trifolium tembense ) in Georgia . Plant Disease , 93 , 202. [ PubMed ] [ Google Scholar ]
- Batuman, O. , Rojas, M. , Almanzar, A. and Gilbertson, R. (2014) First report of Tomato chlorotic spot virus in processing tomatoes in the Dominican Republic . Plant Disease , 98 , 286. [ PubMed ] [ Google Scholar ]
- Batuman, O. , Turini, T.A. , Oliveira, P.V. , Rojas, M.R. , Macedo, M. , Mellinger, H.C . et al (2017) First report of a resistance‐breaking strain of Tomato spotted wilt virus infecting tomatoes with the Sw‐ 5 tospovirus‐resistance gene in California . Plant Disease , 101 , 637. [ Google Scholar ]
- Beaudoin, A.P.L. (2011) Temporal and spatial patterns of thrips dispersal in relation to the epidemiology of tomato spotted wilt virus . PhD Dissertation, Order No. 3497228, North Carolina State University.
- Ben Moussa, A. , Marrakchi, M. and Makni, M. (2005) Characterisation of tospovirus in vegetable crops in Tunisia . Infection Genetics and Evolution , 5 , 312–322. [ Google Scholar ]
- Berndt, O. , Meyhofer, R. and Poehling, H.M. (2004) The edaphic phase in the ontogenesis of Frankliniella occidentalis and comparison of Hypoaspis miles and Hypoaspis aculeifer as predators of soil‐dwelling thrips stages . Biological Control , 30 , 17–24. [ Google Scholar ]
- Bezerra, I.C. , Resende, R. , Pozzer, L. , Nagata, T. , Kormelink, R. and De Ávila, A.C. (1999) Increase of tospoviral diversity in Brazil with the identification of two new tospovirus species, one from chrysanthemum and one from zucchini . Phytopathology , 89 , 823–830. [ PubMed ] [ Google Scholar ]
- Bielza, P. (2008) Insecticide resistance management strategies against the western flower thrips, Frankliniella occidentalis . Pest Management Science , 64 , 1131–1138. [ PubMed ] [ Google Scholar ]
- Bielza, P. (2010) Insecticide resistance management strategies against the western flower thrips, Frankliniella occidentalis . Pest Management Science , 64 , 1131–1138. [ PubMed ] [ Google Scholar ]
- Birithia, R. , Subramanian, S. , Villinger, J. , Muthomi, J.W. , Narla, R.D. and Pappu, H.R. (2012) First report of Tomato yellow ring virus ( Tospovirus , Bunyaviridae ) infecting tomato in Kenya . Plant Disease , 96 , 1384. [ PubMed ] [ Google Scholar ]
- Blystad, D.R. , Naess, V. and Haugslien, S. (1995) Optimizing immunosorbent electron microscopy for detection of pelargonium flower‐break carmovirus in pelargonium . EPPO Bulletin , 25 , 239–245. [ Google Scholar ]
- Boari, A.J. , Maciel‐zambolim, E. , Lau, D.D. , Lima, G.S.A. , Kitajima, E.W. , Brommonschenkel, S.S.H . et al (2002) Detection and partial characterization of an isolate of Groundnut ringspot virus in Solanum sessiliflorum . Fitopatologia Brasileira , 27 , 249–253. [ Google Scholar ]
- Boiteux, L. , Nagata, T. , Dusi, A. and de Avila, A. (1993) Natural occurrence of the two tospovirus species infecting Capsicum spp. in Brazil . Capsicum Eggplant Newsleter , 12 , 75. [ Google Scholar ]
- Bouwen, I. and Maat, D.Z. (1992) Pelargonium flower‐break and pelargonium line pattern viruses in the Netherlands; purification, antiserum preparation, serological identification, and detection in pelargonium by ELISA . Netherlands Journal ofPlant Pathology , 98 , 141–156. [ Google Scholar ]
- Brittlebank, C. (1919) Tomato diseases . Journal of Agricultural Victoria , 27 , 231–235. [ Google Scholar ]
- Brodbeck, B.V. , Stavisky, J. , Funderburk, J.E. , Andersen, P.C. and Olson, S.M. (2001) Flower nitrogen status and populations of Frankliniella occidentalis feeding on Lycopersicon esculentum . Entomologia Experimentalis et Applicata , 99 , 165–172. [ Google Scholar ]
- Broughton, S. , Cousins, D.A. and Rahman, T. (2015) Evaluation of semiochemicals for their potential application in mass trapping of Frankliniella occidentalis (Pergande) in roses . Crop Protection , 67 ,130–135. [ Google Scholar ]
- Buitenhuis, R. and Shipp, J.L. (2005) Efficacy of entomopathogenic nematode Steinernema feltiae (Rhabditida: Steinernematidae) as influenced by Frankliniella occidentalis (Thysanoptera: Thripidae) developmental stage and host plant stage . Journal of Economic Entomology , 98 , 1480–1485. [ PubMed ] [ Google Scholar ]
- Cabanas, D. , Watanabe, S. , Higashi, C.H.V. and Bressan, A. (2013) Dissecting the mode of maize chlorotic mottle virus transmission (Tombusviridae: Machlomovirus) by Frankliniella williamsi (Thysanoptera: Thripidae) . Journal of Economic Entomology , 106 , 16–24. [ PubMed ] [ Google Scholar ]
- Caciagli, P. , Boccardo, G. and Lovisolo, O. (1989) Parietaria mottle virus, a possible new ilarvirus from Parietaria officinalis (Urticaceae) . Plant Pathology , 38 , 577–584. [ Google Scholar ]
- Cai, J.H. , Qin, B.X. , Wei, X.P. , Huang, J. , Zhou, W.L. , Lin, B.S . et al (2011) Molecular identification and characterization of Tomato zonate spot virus in tobacco in Guangxi, China . Plant Disease , 95 , 1483. [ PubMed ] [ Google Scholar ]
- Camelo‐Garca, V.M. , Lima, E.F.B , Mansilla‐Crdova, P.J. , Rezende, J.A.M. , Kitajima, E.W. and Barreto, M. (2014) Occurrence of Groundnut ringspot virus on Brazilian peanut crops . Journal of General Plant Pathology , 80 , 282–286. [ Google Scholar ]
- Castillo‐Loayza, J. (1977) Maize virus and virus‐like diseases in Peru . Proceedings of International Maize Virus Disease Colloquim Workshop (eds. L.E. Williams, D.T. Gordon & L.R. Nault) pp. 40–44.
- Chamberlain, E. and Taylor, G. (1936) The occurrence of spotted‐wilt on tomatoes in New Zealand . New Zealand Journal of Agriculture , 52 , 9–17. [ Google Scholar ]
- Chamberlain, E. and Taylor, G. (1938) Spotted wilt. Host range and transmission by thrips . New Zealand Journal of Science and Technology, Section A. , 20 , 133–142. [ Google Scholar ]
- Chatzivassiliou, E.K. , Weekes, R. , Morris, J. , Wood, K.R. , Barker, I. and Katis, N.I. (2000a) Tomato spotted wilt virus (TSWV) in Greece: its incidence following the expansion of Frankliniella occidentalis , and characterisation of isolates collected from various hosts . Annual of Appliled Biology , 137 , 127–134. [ Google Scholar ]
- Chatzivassiliou, E.K. , Livieratos, I. , Jenser, G. and Katis, N. (2000b) Ornamental plants and thrips populations associated with tomato spotted wilt virus in Greece . Phytoparasitica , 28 , 257–264. [ Google Scholar ]
- Chatzivassilou, E.K. , Livieratos, I. , Katis, N. , Avegelis, A. and Lykouressis, D. (1996) Occurrence of tomato spotted wilt virus in vegetables and ornamentals in Greece . Acta Horticulturae , 431 , 44–50. [ Google Scholar ]
- Chen, L. , Jiao, Z. , Liu, D. , Liu, X. , Xia, Z. , Deng, C . et al (2017) One‐step reverse transcription loop‐mediated isothermal amplification for the detection of Maize chlorotic mottle virus in maize . Journal of Virological Methods , 240 , 49–53. [ PubMed ] [ Google Scholar ]
- Choi, G.S. , Kim, J.S. , Choi, J.K. and Kim, J.H. (2004) Characterization of Tomato spotted wilt virus from paprika in Korea . Plant Pathology Journal , 20 , 297–301. [ Google Scholar ]
- Choi, S.K. and Choi, G.S. (2015) First report of Tomato spotted wilt virus in Solanum tuberosum in Korea . Plant Disease , 99 , 1657. [ PubMed ] [ Google Scholar ]
- Chow, A. , Chau, A. and Heinz, K.M. (2012) Reducing fertilization: a management tactic against western flower thrips on roses . Journal of Applied Entomology , 136 , 520–529. [ Google Scholar ]
- Cloyd, R.A. (2009) Western flower thrips ( Frankliniella occidentalis ) management on ornamental crops grown in greenhouses: have we reached an impasse? Pest Technology , 3 , 1–9. [ Google Scholar ]
- Colariccio, A. , Chaves, A. , Eiras, M. and Chagas, C. (2001a) Identification of the tomato chlorotic spot virus in endive ( Cichorium endiva L.) . Summa Phytopathology , 27 , 325–327. [ Google Scholar ]
- Colariccio, A. , Eiras, M. , Alexandre, L. , Chaves, R. and Chagas, C. (2001b) Characterization of tomato chlorotic spot virus from hydroponic grown lettuce in Brazil . Thrips and Tospoviruses: Proceedings of the 7th International Symposium on Thysanoptera (eds. R. Marullo & L. Mound), pp. 99–104. Reggio Calabria, Italy: ANIC.
- Cook, S.M. , Khan, Z.R. and Pickett, J.A. (2006) The use of push‐pull strategies in integrated pest management . Annual Review of Entomology , 52 , 375–400. [ PubMed ] [ Google Scholar ]
- Cota, E. and Merkuri, J. (2004) Introduction of Frankliniella occidentalis and occurrence of Tomato spotted wilt tospovirus in Albania . EPPO Bulletin , 34 , 421–422. [ Google Scholar ]
- Cox, D. (2017) Quarantine and pre‐shipment uses of methyl bromide 2013–2016 and the potential for its replacement . Report to the Australian Government Department of the Environment and Energy .
- Crosslin, J.M. , Mallik, I. and Gudmestad, N.C. (2009) First report of Tomato spotted wilt virus causing potato tuber necrosis in Texas . Plant Disease , 93 , 845. [ PubMed ] [ Google Scholar ]
- Culbreath, A.K. , Csinos, A.S. , Bertrand, P.F. and Demski, J.W. (1991) Tomato spotted wilt virus epidemic in flue‐cured tobacco in Georgia . Plant Disease , 75 , 483–485. [ Google Scholar ]
- Dal Bio, E. , Chiarrone, G. , Rolleri, J. and Ronc, L. (2001) New tospoviruses found in La Plata . Revista de la Facultad Agronnomia (La Plata) , 104 , 35–40. [ Google Scholar ]
- De Avila, A.C. , De Haan, P. , Kormelink, R. , De Resende, O.R. , Goldbach, R.W. and Peters, D. (1993) Classification of tospoviruses based on phylogeny of nucleoprotein gene sequences . Journal of General Virology , 74 , 153–159. [ PubMed ] [ Google Scholar ]
- Díaz‐Pérez, J.C. and Pappu, H.R. (2000) First report of Tomato spotted wilt virus infection of tomatillo in Georgia . Plant Disease , 84 , 1155. [ PubMed ] [ Google Scholar ]
- Dietzgen, R. , Mann, K. and Johnson, K. (2016) Plant virus–insect vector interactions: current and potential future research directions . Viruses , 8 , 303. [ PMC free article ] [ PubMed ] [ Google Scholar ]
- De Avila, A.C. , Huguenot, C. , De Resende, O.R. , Kitajima, E.W. , Goldbach, R.W. and Peters, D. (1990) Serological differentiation of 20 isolates of tomato spotted wilt virus . Journal of General Virology , 71 , 2801–2807. [ PubMed ] [ Google Scholar ]
- Deewatthanawong, R. , Chanapan, S. and Suwanagul, A. (2016) Evaluation of methyl bromide alternatives to control thrips in orchid cut‐flowers . I International Symposium on Tropical and Subtropical Ornamentals 1167, pp. 393–398.
- Del Ponte, E.M. , Pethybridge, S.J. , Bock, C.H. , Michereff, S.J. , Machado, F.J. and Spolti, P. (2017) Standard area diagram aids for aiding severity estimation: Scientometrics, pathosystems and methodological trends in the last 25 years . Phytopathology , 107 , 1161–1174. [ PubMed ] [ Google Scholar ]
- Del Ponte, E.M. , Nelson, S.C. and Pethybridge, S.J. (2019) Evaluation of app‐embedded disease scales for aiding visual severity estimation of cercospora leaf spot of Table Beet . Plant Disease , 103 , 1347–1356. [ PubMed ] [ Google Scholar ]
- Demirozer, O. , Tyler‐Julian, K. , Funderburk, J. , Norm, L. and Stuart, R. (2012) Frankliniella occidentalis (Pergande) integrated pest management programs for fruiting vegetables in Florida . Pest Management Science , 68 , 1537–1545. [ PubMed ] [ Google Scholar ]
- Deng, T.C. , Chou, C.M. , Chen, C.T. , Tsai, C.H. and Lin, F.C. (2014) First report of Maize chlorotic mottle virus on sweet corn in Taiwan . Plant Disease , 98 , 1748. [ PubMed ] [ Google Scholar ]
- Dikova, B. , Petrov, N. , Djourmanski, A. and Lambev, H. (2013) First report of Tomato spotted wilt virus on a new host Leuzea carthamoides in Bulgaria and the World . Plant Disease , 97 , 1258. [ PubMed ] [ Google Scholar ]
- Dong, J. , Zhang, Z. , Yin, Y. , Cheng, X. , Ding, M. and Fang, Q. (2010) Natural host ranges of Tomato zonate spot virus in Yunnan . Journal of Insect Science , 10 , 12–13. [ Google Scholar ]
- Dong, J.H. , Cheng, X.F. , Yin, Y.Y. , Fang, Q. , Ding, M. , Li, T.T . et al (2008) Characterization of tomato zonate spot virus, a new tospovirus in China . Archives of Virology , 153 , 855–864. [ PubMed ] [ Google Scholar ]
- Ebssa, L. , Borgemeister, C. and Poehling, H.M. (2004) Effectiveness of different species/strains of entomopathogenic nematodes for control of western flower thrips ( Frankliniella occidentalis ) at various concentrations, host densities and temperatures . Biological Control , 29 , 145–154. [ Google Scholar ]
- Eiras, M. , Chaves, A. , Colariccio, A. , Harakava, R. , de Araujo, J. and Chagas, C. (2002) Characterization of a Tomato chlorotic spot virus isolated from gilo in Peraiba Valley, Sao Paulo, Brazil . Fitopatologia Brasileira , 27 , 285–291. [ Google Scholar ]
- EPPO (2004) Data sheets on quarantine pests: Tomato spotted wilt tospovirus . Data Sheets Quar. Pests (revision Orig. 1997c data sheet). [ Google Scholar ]
- Estévez de Jensen, C. and Adkins, S. (2014) First report of Tomato chlorotic spot virus in lettuce in Puerto Rico . Plant Disease , 98 , 1015. [ PubMed ] [ Google Scholar ]
- Estévez de Jensen, C. , Rivera‐Vargas, L.I. , Rodrigues, J.C.V. , Mercado, W. , Frantz, G. , Mellinger, H.C . et al (2013) First report of Tomato chlorotic spot virus (TCSV) in tomato, pepper, and jimsonweed in Puerto Rico . Plant Health Progress , 10.1094/PHP-2013-0812-01-BR [ CrossRef ] [ Google Scholar ]
- Fletcher, J. , France, C. and Butler, R. (2005) Virus surveys of lettuce crops and management of lettuce big‐vein disease in New Zealand . New Zealand Plant Protection , 58 , 239–244. [ Google Scholar ]
- Funderburk, J. (2009) Management of the western flower thrips (Thysanoptera: Thripidae) in fruiting vegetables . Florida Entomologist , 92 , 1–6. [ Google Scholar ]
- Galipienso, L. , Herranz, M.C. , Pallás, V. and Aramburu, J. (2005) Detection of a tomato strain of Parietaria mottle virus (PMoV‐T) by molecular hybridization RT‐PCR in field samples from north‐eastern Spain . Plant Pathology , 54 , 29–35. [ Google Scholar ]
- Gao, Y. , Lei, Z. and Reitz, S.R. (2012a) Western flower thrips resistance to insecticides: detection, mechanisms and management strategies . Pest Management Science , 68 , 1111–1121. [ PubMed ] [ Google Scholar ]
- Gao, Y.L. , Reitz, S.R. , Wang, J. , Tamez‐Guerra, P. , Wang, E.D. , Xu, X.N . et al (2012b) Potential use of the fungus Beauveria bassiana against the western flower thrips Frankliniella occidentalis without reducing the effectiveness of its natural predator Orius sauteri (Hemiptera: Anthocoridae) . Biocontrol Science and Technology , 22 , 803–812. [ Google Scholar ]
- Gera, A. , Kritzman, A. and Cohen, J. (2000a) Pittosporum tobira : a new host for Tomato spotted wilt virus . Plant Disease , 84 , 491. [ PubMed ] [ Google Scholar ]
- Gera, A. , Kritzman, A. , Cohen, J. , Raccah, B. and Antignus, Y. (2000b) Tospoviruses infecting vegetable crops in Israel . EPPO Bulletin , 30 , 289–292. [ Google Scholar ]
- Gilbertson, R.L. , Batuman, O. , Webster, C.G. and Adkins, S. (2015) Role of the insect supervectors Bemisia tabaci and Frankliniella occidentalis in the emergence and global spread of plant viruses . Annual Review of Virology , 2 , 67–93. [ PubMed ] [ Google Scholar ]
- Golnaraghi, A.R. , Shahraeen, N. , Pourrahim, R. , Ghorbani, S. and Farzadfar, S. (2001) First report of Tomato spotted wilt virus on soybean in Iran . Plant Disease , 85 , 1290. [ PubMed ] [ Google Scholar ]
- Gouli, V.V. , Gouli, S.Y. , Skinner, M. and Shternshis, M.V. (2009) Effect of the entomopathogenic fungi on mortality and injury level of western flower thrips, Frankliniella occidentalis . Archives of Phytopathology and Plant Protection , 42 ( 2 ), 118–123. [ Google Scholar ]
- Gowda, M. , Das, B. , Makumbi, D. , Babu, R. , Semagn, K. , Mahuku, G . et al (2015) Genome‐wide association and genomic prediction of resistance to maize lethal necrosis disease in tropical maize germplasm . Theoretical and Applied Genetics , 128 , 1957–1968. [ PMC free article ] [ PubMed ] [ Google Scholar ]
- Gracia, O. , De Borbon, C.M. , Granval De Millan, N. and Cuesta, G.V. (1999) Occurrence of different tospoviruses in vegetable crops in Argentina . Journal of Phytopathology , 147 , 223–227. [ Google Scholar ]
- Groves, R.L. , Kennedy, G.G. , Walgenbach, J.F. and Moyer, J.W. (1998) Inoculation of Tomato spotted wilt virus into Cotton . Plant Disease , 82 , 959. [ PubMed ] [ Google Scholar ]
- Haliwell, R. and Philley, G. (1974) Spotted wilt of peanut in Texas . Plant Disease Report , 58 , 23–25. [ Google Scholar ]
- Hassani‐Mehraban, A. , Botermans, M. , Verhoeven, J.T.J. , Meekes, E. , Saaijer, J. , Peters, D . et al (2010) A distinct tospovirus causing necrotic streak on Alstroemeria sp. in Colombia . Archives of Virology , 155 , 423–428. [ PMC free article ] [ PubMed ] [ Google Scholar ]
- Hassani‐Mehraban, A. , Saaijer, J. , Peters, D. , Goldbach, R. and Kormelink, R. (2005) A new tomato‐infecting Tospovirus from Iran . Phytopathology , 95 , 852–858. [ PubMed ] [ Google Scholar ]
- Hassani‐Mehraban, A. , Saaijer, J. , Peters, D. , Goldbach, R. and Kormelink, R. (2007) Molecular and biological comparison of two tomato yellow ring virus (TYRV) isolates: challenging the tospovirus species concept . Archievs of Virology , 152 , 85–96. [ PubMed ] [ Google Scholar ]
- Healey, M.A. , Senior, L.J. , Brown, P.H. and Duff, J. (2017) Relative abundance and temporal distribution of adult Frankliniella occidentalis (Pergande) and Frankliniella schultzei (Trybom) on French bean, lettuce, tomato and zucchini crops in relation to crop age . Journal of Asia‐Pacific Entomology , 20 , 859–865. [ Google Scholar ]
- Herron, G.A. , Gunning, R.V. , Cottage, E.L. , Borzatta, V. and Gobbi, C. (2014) Spinosad resistance, esterase isoenzymes and temporal synergism in Frankliniella occidentalis (Pergande) in Australia . Pesticide Biochemistry and Physiology , 114 , 32–37. [ PubMed ] [ Google Scholar ]
- Hogenhout, S.A. , Ammar, E.D. , Whitfield, A.E. and Redinbaugh, M.G. (2008) Insect vector interactions with persistently transmitted viruses . Annual Review of Phytopathology , 46 , 327–359. [ PubMed ] [ Google Scholar ]
- Holcomb, G.E. and Valverde, R.A. (2000) First report of Oidium sp. powdery mildew and Tomato spotted wilt virus on Melampodium divaricatum . Plant Disease , 84 , 1152–1152. [ PubMed ] [ Google Scholar ]
- Holcomb, G.E. , Valverde, R.A. , Sim, J. and Nuss, J. (1999) First report on natural occurrence of Tomato spotted wilt tospovirus in Basil ( Ocimum basilicum ) . Plant Disease , 83 , 966. [ PubMed ] [ Google Scholar ]
- Holguín‐Peña, R.J. and Rueda‐Puente, E.O. (2007) Detection of Tomato spotted wilt virus in tomato in the Baja California Peninsula of Mexico . Plant Disease , 91 , 1682. [ PubMed ] [ Google Scholar ]
- Houle, J.L. and Kennedy, G.G. (2017) Tomato spotted wilt virus can infect resistant tomato when western flower thrips inoculate blossoms . Plant Diseae , 101 , 1666–1670. [ PubMed ] [ Google Scholar ]
- Huang, C.J. , Liu, Y. , Yu, H.Q. and Li, B.L. (2015) Occurrence of Tomato zonate spot virus on potato in China . Plant Disease , 99 , 733. [ PubMed ] [ Google Scholar ]
- Huang, K.S. , Lee, S.E. , Yeh, Y. , Shen, G.S. , Mei, E. and Chang, C.M. (2010) Taqman real‐time quantitative PCR for identification of western flower thrip ( Frankliniella occidentalis ) for plant quarantine . Biology letters , 6 ( 4 ), 555–557. [ PMC free article ] [ PubMed ] [ Google Scholar ]
- Ivars, P. , Alonso, M. , Borja, M. and Hernández, C. (2004) Development of a non‐radioactive dot‐blot hybridisation assay for the detection of Pelargonium flower break virus and Pelargonium line pattern virus . Euroupean Journal of Plant Pathology , 110 , 275–283. [ Google Scholar ]
- Janssen, D. , Saez, E. , Segundo, E. , Martín, G. , Gil, F. and Cuadrado, I.M. (2005) Capsicum annuum – a new host of Parietaria mottle virus in Spain . Plant Pathology , 54 , 567. [ Google Scholar ]
- Jiang, X. , Wilkinson, D. and Berry, J. (1990) An outbreak of maize chlorotic mottle virus in Hawaii and possible association with thrips . Phytopathology , 80 , 1060. [ Google Scholar ]
- Jones, D.R. (2005) Plant viruses transmitted by thrips . Euroupean Journal Plant Pathology , 113 , 119–157. [ Google Scholar ]
- Jorda, C. (1993) Nuevas virosis de mayor incidencia en cultivos horticolas . Phytoma Espana , 50 , 7–13. [ Google Scholar ]
- Karavina, C. , Ibaba, J.D. and Gubba, A. (2016a) First report of Tomato spotted wilt virus infecting butternut squash ( Cucurbita moschata ) in Zimbabwe . Plant Disease , 100 , 870. [ Google Scholar ]
- Karavina, C. , Ximba, S. , Ibaba, J.D. and Gubba, A. (2016b) First report of a mixed infection of Potato virus Y and Tomato spotted wilt virus on pepper ( Capsicum annuum ) in Zimbabwe . Plant Disease , 100 , 1513. [ Google Scholar ]
- Kigathi, R. and Poehling, H.M. (2012) UV‐absorbing films and nets affected the dispersal of western flower thrips, Frankliniella occidentalis (Thysanoptera: Thripidae) . Journal of Applied Entomology , 136 , 761–771. [ Google Scholar ]
- Kirk, W.D.J. and Terry, L.I. (2003) The spread of the western flower thrips Frankliniella occidentalis (Pergande) . Agricultural and Forest Entomology , 5 , 301–310. [ Google Scholar ]
- Kirk, W.D. (2017) The aggregation pheromones of thrips (Thysanoptera) and their potential for pest management . International Journal of Tropical Insect Science , 37 , 41–49. [ Google Scholar ]
- Kisten, L. , Moodley, V. , Gubba, A. and Mafongoya, P.L. (2016) First detection of Tomato spotted wilt virus (TSWV) on Amaranthus thunbergii in South Africa . Plant Disease , 100 , 2176. [ Google Scholar ]
- Kliot, A. , Kontsedalov, S. , Lebedev, G. and Ghanim, M. (2016) Advances in whiteflies and thrips management Advances in Insect Control and Resistance Management . pp. 205–218. Springer, Cham. [ Google Scholar ]
- Koehler, A.M. , Brown, J.A. , Huber, B. , Wehner, T.C. and Shew, H.D. (2016) First report of Tomato spotted wilt virus in Stevia rebaudiana in North Carolina . Plant Disease , 100 , 1251. [ Google Scholar ]
- Krczal, G. , Albouy, J. , Damy, I. , Kusiak, C. , Deogratias, J.M. , Moreau, J.P . et al (1995) Transmission of pelargonium flower break virus (PFBV) in irrigation systems and by thrips . Plant Disease , 79 , 163–166. [ Google Scholar ]
- Kucharek, T. , Brown, L. , Johnson, F. and Funderburk, J. (2000) Tomato spotted wilt virus of agronomic, vegetable, and ornamental crops Plant Pathology Fact Sheet Circ‐914 ., pp. 13 Florida Cooperative Extension Service/Institute of Food and Agricultural Sciences/University of Florida. [ Google Scholar ]
- Kuo, Y.W. , Gilbertson, R.L. , Turini, T. , Brennan, E.B. , Smith, R.F. and Koike, S.T. (2014) Characterization and epidemiology of outbreaks of Impatiens necrotic spot virus on lettuce in coastal California . Plant Disease , 98 , 1050–1059. [ PubMed ] [ Google Scholar ]
- Kusia, E.S. , Subramanian, S. , Nyasani, J.O. , Khamis, F. , Villinger, J. , Ateka, E.M . et al (2015) First report of lethal necrosis disease associated with co‐infection of finger millet with Maize chlorotic mottle virus and Sugarcane mosaic virus in Kenya . Plant Disease , 99 , 899. [ Google Scholar ]
- Latham, L.J. and Jones, R.A.C. (1997) Occurrence of tomato spotted wilt tospovirus in native flora, weeds, and horticultural crops . Australian Journal of Agricultural Research , 48 , 359. [ Google Scholar ]
- Leão, E.U. , de Almeida Spadotti, D.M. , Rocha, K.C.G. , de Fátima da CunhaPantoja, K. , Rezende, J.A.M. , Pavan, M.A . et al (2015) Citrullus lanatus is a new natural host of Groundnut ringspot virus in Brazil . Journal of Phytopathology , 163 , 1014–1018. [ Google Scholar ]
- Lebas, B.S. and Ochoa‐Corona, F. (2007) Impatiens necrotic spot virus. Characterization, Diagnosis and Management of Plant Viruses. Vol. 4: Grain Crops and Ornamentals (eds. Rao G.P., Bragard C. & Lebas B.S.M.), pp. 221–243. Texas: Stadium Press LLC. [ Google Scholar ]
- Lebas, B.S. , Ochoa‐Corona, F. , Elliott, D. , Tang, Z. , Alexander, B.J. and Froud, K.J. (2004) An investigation of an outbreak of Impatiens necrotic spot virus in New Zealand . Phytopathology , 94 , S57–S58. [ Google Scholar ]
- Lee, S.J. , Kim, S. , Kim, J.C. , Lee, M.R. , Hossain, M. , Shin, T.S . et al (2017) Entomopathogenic Beauveria bassiana granules to control soil‐dwelling stage of western flower thrips, Frankliniella occidentalis (Thysanoptera: Thripidae) . BioControl , 62 , 639–648. [ Google Scholar ]
- Li, D.G. , Shang, X.Y. , Reitz, S.R. , Nauen, R. , Lei, Z.R. , Lee, S.H . et al (2016) Field resistance to spinosad in western flower thrips Frankliniella occidentalis (Thysanoptera: Thripidae) . Journal of Integrative Agriculture , 15 , 2803–2808. [ Google Scholar ]
- Li, Y.Y. , Xiao, L. , Tan, G.L. , Fu, X.P. , Li, R.H. and Li, F. (2015) First report of Tomato spotted wilt virus on celery in China . Plant Disease , 99 , 734. [ Google Scholar ]
- Lima, M.F. , de Ávila, A.C. , da G Wanderley, L.J. , Nagata, T. and da Gama, L.J.W. (1999) Coriander: a new natural host of Groundnut ring spot virus in Brazil . Plant Disease , 83 , 878. [ PubMed ] [ Google Scholar ]
- Liu, L.Y. , Ye, H.Y. , Chen, T.H. and Chen, T.C. (2017) Development of a microarray for simultaneous detection and differentiation of different tospoviruses that are serologically related to Tomato spotted wilt virus . Journal of Virology , 14 , 1. [ PMC free article ] [ PubMed ] [ Google Scholar ]
- Liu, Y. , Huang, C.J. , Tao, X.R. and Yu, H.Q. (2015) First report of Tomato zonate spot virus in Iris tectorum in China . Plant Disease , 99 , 164. [ PubMed ] [ Google Scholar ]
- Londoño, A. , Capobianco, H. , Zhang, S. and Polston, J.E. (2012) First record of Tomato chlorotic spot virus in the USA . Tropical Plant of Pathology , 37 , 333–338. [ Google Scholar ]
- Louro, D. (1996) Detection and identification of tomato spotted wilt virus and impatiens necrotic spot virus in Portugal . Acta Horticulturae , 431 , 99–105. [ Google Scholar ]
- Lukanda, M. , Owati, A. , Ogunsanya, P. , Valimunzigha, K. , Katsongo, K. , Ndemere, H . et al (2014) First report of Maize chlorotic mottle virus infecting maize in the Democratic Republic of the Congo . Plant Disease , 98 , 1448. [ PubMed ] [ Google Scholar ]
- Mahuku, G. , Lockhart, B.E. , Wanjala, B. , Jones, M.W. , Kimunye, J.N. , Stewart, L.R . et al (2015) Maize lethal necrosis (MLN), an emerging threat to maize‐based food security in Sub‐Saharan Africa . Phytopathology , 105 , 956–965. [ PubMed ] [ Google Scholar ]
- Maluf, W. , Toma‐Brahini, M. and Corte, R.D. (1991) Progress in breeding tomatoes for resistance to tomato spotted wilt . Brazilian Journal of Genetics , 14 , 509–525. [ Google Scholar ]
- Maniania, N.K. , Ekesi, S. , Löhr, B. and Mwangi, F. (2003) Prospects for biological control of the western flower thrips, Frankliniella occidentalis , with the entomopathogenic fungus, Metarhizium anisopliae , on chrysanthemum . Mycopathologia , 155 , 229–235. [ PubMed ] [ Google Scholar ]
- Marchoux, G. , Gebre‐selassie, K. and Villevieille, M. (1991) Detection of tomato spotted wilt virus and transmission by Frankliniella occidentalis in France . Plant Pathology , 40 , 347–351. [ Google Scholar ]
- Martínez, R.T. , Poojari, S. , Tolin, S.A. , Cayetano, X. and Naidu, R.A. (2014) First report of Tomato spotted wilt virus in peppers and tomato in the Dominican Republic . Plant Disease , 98 , 163. [ PubMed ] [ Google Scholar ]
- Martinez, D.L.O. , Zavaleta‐Mejía, E. , Mora‐Aguilera, G. and Johansen N.R.M. (1999) Implications of weed composition and thrips species for the epidemiology of tomato spotted wilt in chrysanthemum ( Dendranthema grandiflora ) . Plant Pathology , 48 , 707–717. [ Google Scholar ]
- Marys, E. , Mejías, A. , Rodríguez‐Román, E. , Avilán, D. , Hurtado, T. , Fernández, A. et al (2014) The first report of Tomato spotted wilt virus on gerbera and chrysanthemun in Venezuela . Plant Disease , 98 , 1161. [ PubMed ] [ Google Scholar ]
- Massumi, H. , Samei, A. , Pour, A.H. , Shaabanian, M. and Rahimian, H. (2007) Occurrence, distribution, and relative incidence of seven viruses infecting greenhouse‐grown cucurbits in Iran . Plant Disease , 91 , 159–163. [ PubMed ] [ Google Scholar ]
- Massumi, H. , Shaabanian, M. , Pour, A.H. , Heydarnejad, J. and Rahimian, H. (2009) Incidence of viruses infecting tomato and their natural hosts in the southeast and central regions of Iran . Plant Disease , 93 , 67–72. [ PubMed ] [ Google Scholar ]
- Mavric, I. and Ravnikar, M. (2001) First report of Tomato spotted wilt virus and Impatiens necrotic spot virus in Slovenia . Plant Disease , 85 , 1288. [ PubMed ] [ Google Scholar ]
- Messelink, G.J. and Janssen, A. (2014) Increased control of thrips and aphids in greenhouses with two species of generalist predatory bugs involved in intraguild predation . Biological Control , 79 , 1–7. [ Google Scholar ]
- Messelink, G.J. , van Steenpaal, S.E.F. and Ramakers, P.M.J. (2006) Evaluation of phytoseiid predators for control of western flower thrips on greenhouse cucumber . BioControl , 51 , 753–768. [ Google Scholar ]
- Mo, L.F. , Zhi, J.R. and Tian, T. (2013) Biological control efficiency of Orius similis Zheng (Hemiptera: Anthocoridae) on Frankliniella occidentalis (Pergande) under different spatial and caged conditions . Acta Ecologica Sinica , 33 , 7132–7139. [ Google Scholar ]
- Momol, M.T. , Pappu, H.R. , Dankers, W. , Rich, J.R. and Olson, S.M. (2000) First report of Tomato spotted wilt virus in habanero and tabasco peppers in Florida . Plant Disease , 84 , 1154. [ PubMed ] [ Google Scholar ]
- Moore, E. (1933) The kromnek or kat river diease of tobacco and tomato in the east province (South Africa). Deptment of Agriculture, Union of South Africa . Science Bulletin , 123 , 5–28. [ Google Scholar ]
- Moore, E. and Andessen, E.E. (1939) Notes on plant virus diseases in South Africa. the kromnek disease of tobacco and tomato. Department of Agriculture, Union of South Africa . Science Bulletin , 182 , 1–36. [ Google Scholar ]
- Morales, F. , Arroyave, J.A. , Castillo, J. and Leon, C.D. (1999) Cytopathology of Mazie chlorotic mottle virus in Zea mays L . Maydica , 44 , 231–235. [ Google Scholar ]
- Morse, J.G. and Hoddle, M.S. (2006) Invasion biology of thrips . Annual Review of Entomology , 51 , 67–89. [ PubMed ] [ Google Scholar ]
- Mouden, S. , Sarmiento, K.F. , Klinkhamer, P.G. and Leiss, K.A. (2017) Integrated pest management in western flower thrips: past, present and future . Pest Management Science , 73 , 813–822. [ PMC free article ] [ PubMed ] [ Google Scholar ]
- Mound, L.A. (2002) So many thrips–so few tospoviruses//Thrips and tospoviruses . Proceedings of the 7th International Symposium on Thysanoptera . Australian National Insect Collection, Canberra, 15–18.
- Mound, L.A. (2004) Thysanoptera: diversity and interactions . Annual Review of Entomology , 50 , 247–269. [ PubMed ] [ Google Scholar ]
- Moussa, A. Ben , Makni, M. and Marrakchi, M. (2000) Identification of the principal viruses infecting tomato crops in Tunisia . EPPO Bulletin , 30 , 293–296. [ Google Scholar ]
- Mullis, S.W. , Csinos, A.S. , Gitaitis, R.D. and Martinez‐Ochoa, N. (2006) First report of pinaceae in Georgia naturally infected with Tomato spotted wilt virus . Plant Disease , 90 , 376. [ PubMed ] [ Google Scholar ]
- Mullis, S.W. , Langston, D.B. , Gitaitis, R.D. , Sherwood, J.L. , Csinos, A.C. , Riley, D.G . et al (2004) First report of vidalia onion ( Allium cepa ) naturally infected with Tomato spotted wilt virus and Iris yellow spot virus (family Bunyaviridae , genus Tospovirus ) in Georgia . Plant Disease , 88 , 1285. [ PubMed ] [ Google Scholar ]
- Mumford, R.A. , Barker, I. and Wood, K.R. (1996) The biology of the tospoviruses . Annals of Applied Biology , 128 , 159–183. [ Google Scholar ]
- Mumford, R.A. , Jarvis, B. , Morris, J. and Blockley, A. (2003) First report of Chrysanthemum stem necrosis virus (CSNV) in the UK . Plant Pathology , 52 , 779. [ Google Scholar ]
- Nagata, T. , Almeida, A.C.L. , Resende, R.O. and de Avila, A.C. (2004) The competence of four thrips species to transmit and replicate four tospoviruses . Plant Pathology , 53 , 136–140. [ Google Scholar ]
- Naidu, R.A. , Deom, C.M. and Sherwood, J.L. (2001) First report of Frankliniella fusca as a vector of Impatiens necrotic spot tospovirus . Plant Disease , 85 , 1211. [ PubMed ] [ Google Scholar ]
- Niblett, C. and Clafin, L. (1978) Maize lethal necrosis–a new virus disease of maize in Kansas . Plant Disease Report , 62 , 15–19. [ Google Scholar ]
- Nikolić, D. , Stanković, I. , Vučurović, A. , Ristić, D. , Milojević, K. , Bulajić, A . et al (2013) First report of Tomato spotted wilt virus on Brugmansia sp. in Serbia . Plant Disease , 97 , 850. [ PubMed ] [ Google Scholar ]
- Nischwitz, C. , Mullis, S.W. , Gitaitis, R.D. and Csinos, A.S. (2006a) First report of Tomato spotted wilt virus in Soybean ( Glycine max ) in Georgia . Plant Disease , 90 , 524. [ PubMed ] [ Google Scholar ]
- Nischwitz, C. , Mullis, S.W. , Gitaitis, R.D. , Csinos, A.S. and Olson, S.M. (2006b) First report of Tomato spotted wilt virus in leek ( Allium porrum ) in the United States . Plant Disease , 90 , 525. [ PubMed ] [ Google Scholar ]
- Northfield, T.D. , Paini, D.R. , Funderburk, J.E. and Reitz, S.R. (2008) Annual cycles of Frankliniella spp. (Thysanoptera: Thripidae) thrips abundance on North Florida uncultivated reproductive hosts: predicting possible sources of pest outbreaks . Annals of the Entomological Society of America , 101 , 769–778. [ Google Scholar ]
- Nyasani, J.O. , Meyhöfer, R. , Subramanian, S. and Poehling, H.M. (2013) Feeding and oviposition preference of Frankliniella occidentalis for crops and weeds in Kenyan French bean fields . Journal of Applied Entomology , 137 , 204–213. [ Google Scholar ]
- Ogada, P.A. and Poehling, H.M. (2015) Sex‐specific influences of Frankliniella occidentalis (western flower thrips) in the transmission of Tomato spotted wilt virus (Tospovirus) . Journal of Plant Disease Protection , 122 , 264–274. [ Google Scholar ]
- Okazaki, S. , Okuda, M. , Komi, K. , Yamasaki, S. , Okuda, S. , Sakurai, T . et al (2011) The effect of virus titre on acquisition efficiency of Tomato spotted wilt virus by Frankliniella occidentalis and the effect of temperature on detectable period of the virus in dead bodies . Australas Plant Pathology , 40 , 120–125. [ Google Scholar ]
- Okazaki, S. , Okuda, M. , Komi, K. , Yoshimatsu, H. and Iwanami, T. (2007) Overwintering viruliferous Frankliniella occidentalis (Thysanoptera: Thripidae) as an infection source of Tomato spotted wilt virus in green pepper Fields . Plant Disease , 91 , 842–846. [ PubMed ] [ Google Scholar ]
- Okuda, M. , Fuji, S. , Okuda, S. , Sako, K. and Iwanami, T. (2010) Evaluation of the potential of thirty two weed species as infection sources of Impatiens necrotic spot virus . Journal of Plant Pathology , 92 , 357–361. [ Google Scholar ]
- Okuda, S. , Okuda, M. , Matsuura, S. , Okazaki, S. and Iwai, H. (2013) Competence of Frankliniella occidentalis and Frankliniella intonsa strains as vectors for Chrysanthemum stem necrosis virus . European Journal of Plant Pathology , 136 , 355–362. [ Google Scholar ]
- Otieno, J.A. , Stukenberg, N. , Weller, J. and Poehling, H.M. (2018) Efficacy of LED‐enhanced blue sticky traps combined with the synthetic lure Lurem‐TR for trapping of western flower thrips ( Frankliniella occidentalis ) . Journal of Pest Science , 91 , 1301–1314. [ Google Scholar ]
- Pérez‐Colmenares, Y. , Mejías, A. , Rodríguez‐Román, E. , Avilán, D. , Gómez, J.C. , Marys, E . et al (2015) Identification of Tomato spotted wilt virus associated with fruit damage during a recent virus outbreak in pepper in Venezuela . Plant Disease , 99 , 896. [ Google Scholar ]
- Pappu, H.R. , Jones, R.A.C. and Jain, R.K. (2009) Global status of tospovirus epidemics in diverse cropping systems: successes achieved and challenges ahead . Virus Reseach , 141 , 219–236. [ PubMed ] [ Google Scholar ]
- Parrella, G. (2002) First report of Parietaria mottle virus in Mirabilis jalapa . Plant Pathology , 51 , 401. [ Google Scholar ]
- Parrella, G. , Greco, B. and Troiano, E. (2016) Severe symptoms of mosaic and necrosis in bell pepper associated with Parietaria mottle virus in Italy . Plant Disease , 100 , 1514. [ Google Scholar ]
- Parrella, G. , Greco, B. and Troiano, E. (2017) First report of Parietaria mottle virus associated with yellowing disease in Diplotaxis tenuifolia in Italy . Plant Disease , 101 , 850. [ Google Scholar ]
- Pearce, M. (2005) 2004 Georgia plant disease loss estimates . University of Georgia , Cooperative Extension Service , 24.
- Pearsall, I.A. , and Myers, J.H. (2000) Population dynamics of western Bower thrips (Thysanoptera: Thripidae) in nectarine orchards in British Columbia . Journal of Economic Entomology , 93 , 264–275. [ PubMed ] [ Google Scholar ]
- Peters, D. , Wijkamp, I. , van De Wetering, F. and Goldbach, R. (1996) Vector relations in the transmission and epidemiology of tospoviruses . Acta Horticulturae , 431 , 29–43. [ Google Scholar ]
- Pittman, H. (1927) Spotted wilt of tomatoes. preliminary note concerning the transmission of the spotted wilt of tomatoes by an insect vector ( Thrips tabaci Lind.) . Australian Council for Scientific and Industrial Research Bulliten , 1 , 74–77. [ Google Scholar ]
- Pourrahim, R. , Farzadfar, S. , Moini, A.A. , Shahraeen, N. and Ahoonmanesh, A. (2001) First report of tomato spotted wilt virus on potatoes in Iran . Plant Disease , 85 , 442. [ PubMed ] [ Google Scholar ]
- Quito‐Avila, D.F. , Alvarez, R.A. and Mendoza, A.A. (2016) Occurrence of maize lethal necrosis in Ecuador: a disease without boundaries . Eurpean Journal of Plant Pathology , 146 , 705–710. [ Google Scholar ]
- Rabelo, L. , Pedrazzoli, D. , Novaes, Q. , Nagata, T. , Rezende, E. and Kitajima, J. (2002) High incidence of tomato chlorotic spot virus in the state of Sao Paulo, Brazil . Fitopatologia Brasileira , 27 , 105–112. [ Google Scholar ]
- Ramasso, E. , Roggero, P. , Dellavalle, G. and Lisa, V. (1997) Necrosi apicale del pomodoro causata da un Ilarvirus . Mikologiya I Fitopatologiya , 1 , 71–77. [ Google Scholar ]
- Rasoulpour, R. and Izadpanah, K. (2007) Characterisation of cineraria strain of Tomato yellow ring virus from Iran . Australas. Plant Pathology , 36 , 286–294. [ Google Scholar ]
- Ravnikar, M. , Vozelj, N. , Mavriè, I. , Svigelj, S. , Zupanèiè, M. and Petroviè, N. (2003) Detection of Chrysanthemum stem necrosis virus and Tomato spotted wilt virus in chrysanthemum . Abstracts 8th International Congress of Plant Pathology ICPP , Christchurch (NZ).
- Reitz S.R. (2009) Biology and ecology of the western flower thrips (Thysanoptera: Thripidae): the making of a pest . Florida Entomology , 92 , 7–13. [ Google Scholar ]
- Reitz, S.R. , Gao, Y.L. and Lei, Z.R. (2011) Thrips: Pests of concern to China and the United States . Journal of Integrative Agriculture , 10 , 867–892. [ Google Scholar ]
- Ren, J. , Lei, Z.R. and Hua, L. (2008) The study on trapping effects of colorful card on Frankliniella occidentalis (Pergande) . Chinese Plant Protection , 28 , 34–35. [ Google Scholar ]
- Renukadevi, P. , Nagendran, K. , Nakkeeran, S. , Karthikeyan, G. , Jawaharlal, M. , Alice, D . et al (2015) First report of Tomato spotted wilt virus infection of chrysanthemum in India . Plant Disease , 99 , 1190. [ Google Scholar ]
- Resende, R. , Pozzer, L. , Nagata, T. , Bezerra, I. , Lima, M. , Giordano, L . et al (1996) New tospoviruses found in Brazil . Acta Horticulture , 431 , 78–89. [ Google Scholar ]
- Rico, P. and Hernández, C. (2006) Infectivity of in vitro transcripts from a full‐length cDNA clone of perlargonium flower break virus in an experimental and a natural host . Journal of Plant Pathology , 88 , 103–106. [ Google Scholar ]
- Rico, P. , Hernndez, C. and Hernández, C. (2004) Complete nucleotide sequence and genome organization of Pelargonium flower break virus . Archievs Virology , 149 , 641–651. [ PubMed ] [ Google Scholar ]
- Rico, P. , Ivars, P. , Elena, S.F. and Hernandez, C. (2006) Insights into the selective pressures restricting Pelargonium flower break virus genome variability: evidence for host adaptation . Journal of Virology , 80 , 8124–8132. [ PMC free article ] [ PubMed ] [ Google Scholar ]
- Riley, D. , Sparks Jr, A. , Srinivasan, R. , Kennedy, G. , Fonsah, G. , Scott, J. et al (2018) Thrips: Biology, ecology, and management Sustainable Management of Arthropod Pests of Tomato , pp. 49–71. Academic Press. [ Google Scholar ]
- Roggero, P. , Ciuffo, M. , Katis, N. , Alioto, D. , Crescenzi, A. , Parrella, G . et al (2000) Necrotic disease in tomatoes in greece and southern Italy caused by the tomato strain of Parietaria mottle virus . Journal of Plant Pathology , 82 , 159. [ Google Scholar ]
- Rosales, M. , Pappu, H. , Arayam, C. and Aljaro, A. (2007) Characterization of Tomato spotted wilt virus ( Tospovirus , Bunyaviridae ) from lettuce ( Lactuca sativa ) in Chile . Phytopathology , 97 , S101. [ Google Scholar ]
- Rotenberg, D. , Jacobson, A.L. , Schneweis, D.J. and Whitfield, A.E. (2015) Thrips transmission of tospoviruses . Current Opinion of Virology , 15 , 80–89. [ PubMed ] [ Google Scholar ]
- Saito, T. and Brownbridge, M. (2016) Compatibility of soil‐dwelling predators andmicrobial agents and their efficacy in controlling soil‐dwelling stages of western flower thrips Frankliniella occidentalis . Biological Control , 92 , 92–100. [ Google Scholar ]
- Sakurai, T. , Inoue, T. and Tsuda, S. (2004) Distinct efficiencies of Impatiens necrotic spot virus transmission by five thrips vector species (Thysanoptera: Thripidae) of tospoviruses in Japan . Applied Entomology and Zoology , 39 , 71–78. [ Google Scholar ]
- Salamon, P. , Nemes, K. , Salánki, K. and Palkovics, L. (2012) First report of natural infection of pea ( Pisum sativum ) by Tomato spotted wilt virus in Hungary . Plant Disease , 96 , 295. [ PubMed ] [ Google Scholar ]
- Samuel, G. , Bald, J. and Pittman, H. (1930) Investigations on “spotted wilt” of tomatoes . Australian Council for Scientific and Industrial Research , 44 , 1–64. [ Google Scholar ]
- Sarkar, S.C. , Wang, E.D. , Zhang, Z.K. , Wu, S.Y. and Lei, Z.R. (2019) Laboratory and glasshouse evaluation of the green lacewing, Chrysopa pallens (Neuroptera: Chrysopidae) against the western flower thrips, Frankliniella occidentalis (Thysanoptera: Thripidae) . Applied Entomology and Zoology , 10.1007/s13355-018-0601-9 [ CrossRef ] [ Google Scholar ]
- Schneweis, D.J. , Whitfield, A.E. and Rotenberg, D. (2017) Thrips developmental stage‐specific transcriptome response to tomato spotted wilt virus during the virus infection cycle in Frankliniella occidentalis , the primary vector . Virology , 500 , 226–237. [ PubMed ] [ Google Scholar ]
- Schuch, U.K. , Rdak, R.A. and Behtke, J.A. (1998) Cultivar, fertilizer and irrigation effect vegetative growth and susceptibility of chrysanthemum to western flower thrips . Journal of America Society Horticulture Science , 123 , 727–733. [ Google Scholar ]
- Sialer, M.M.F. and Gallitelli, D. (2000) The occurrence of Impatiens necrotic spot virus and Tomato spotted wilt virus in mixed infection in tomato . Journal of Plant Pathology , 82 , 244. [ Google Scholar ]
- Smith, K. (1932) Studies on plant virus diseases XI. Further experiments with a ringspot virus: its identification with spotted wilt of tomato . Annals of Applied Biology , 19 , 305–320. [ Google Scholar ]
- Spadotti, D. , Leão, E. and Rocha, K. (2014) First report of Groundnut ringspot virus in cucumber fruits in Brazil . New Dosease Reports , 5197.
- Stafford, C.A. , Walker, G.P. and Ullman, D.E. (2011) Infection with a plant virus modified vector feeding behavior . Proceedings of the National Academy of Sciences USA , 108, 9350–9355. [ PMC free article ] [ PubMed ]
- Stanković, I. , Bulajić, A. , Vučurović, A. , Ristić, D. , Jović, J. and Krstić, B. (2011) First report of Tomato spotted wilt virus on Gerbera hybrida in Serbia . Plant Disease , 95 , 226. [ PubMed ] [ Google Scholar ]
- Stanković, I. , Bulajić, A. , Vučurović, A. , Ristić, D. , Milojević, K. , Nikolić, D . et al (2012) First report of Tomato spotted wilt virus infecting onion and garlic in Serbia . Plant Disease , 96 , 918. [ PubMed ] [ Google Scholar ]
- Stanković, I. , Bulajić, A. , Vučurović, A. , Ristić, D. , Milojević, K. , Nikolić, D . et al (2013) First report of Tomato spotted wilt virus on chrysanthemum in Serbia . Plant Disease , 97 , 150. [ PubMed ] [ Google Scholar ]
- Stavisky, J. , Funderburk, J.E. , Brodbeck, B.V. , Olson, S.M. and Andersen, P.C. (2002) Population dynamics of Frankliniella spp. and tomato spotted wilt incidence as influenced by cultural management tactics in tomato . Journal of Economic Entomology , 95 , 1216–1221. [ PubMed ] [ Google Scholar ]
- Stone, B.O.M. and Hollings, M. (1973) Some properties of pelargonium flower‐break virus . Annual of Applied Biology , 75 , 15–23. [ Google Scholar ]
- Subbaiah, K.V. , Sai Gopal, D.V.R. and Krishna Reddy, M. (2000) First report of a tospovirus on sunflower ( Helianthus annus L.) from India . Plant Disease , 84 , 1343. [ PubMed ] [ Google Scholar ]
- Szostek, S.A. , Rodriguez, P. , Sanchez, J. , Adkins, S. and Naidu, R.A. (2017) Western flower thrips can transmit Tomato spotted wilt virus from virus‐infected tomato fruits . Plant Health Prog , 18 ( 1 ), 1–6. [ Google Scholar ]
- Terry, L.I. (1997) Host selection, communication and reproductive behavior Thrips as Crop Pests (ed. Lewis T.), pp. 65–118. CAB International, New York. [ Google Scholar ]
- Tinoco, C.E. , Gutiérrez, G.A.M. , Bolaños, T.A. and Sánchez, D.M. (2014) Screen porosity and exclusion of pest in greenhouse tomatoes ( Solanum lycopersicum L.) . Southwest Entomology , 39 , 625–634. [ Google Scholar ]
- Toledo‐Hernández, R.A. , Ortiz‐Girón, J.A. and Sánchez, D. (2017) Preliminary observations on pathogenicity of commercial formulations of Metarhizium anisopliae and Beauveria bassiana entomopathogenic fungi for control of Frankliniella invasor under laboratory conditions . Southwest Entomology , 42 , 1035–1040. [ Google Scholar ]
- Tommasini, M.G. and Maini, S. (1995) Frankliniella occidentalis and other thrips harmful to vegetable and ornamental crops in Europe Biological Control of Thrips (eds. Loomans A.J.L., van Lenteren J.C., Tommasini M.G., Maini S. & Riudavets J.), Papers 95.1, pp. 1–42. Wageningen Agricultural University, the Netherlands. [ Google Scholar ]
- Trkulja, V. , Salapura, J.M. , Ćurković, B. , Stanković, I. , Bulajić, A. , Vučurović, A . et al (2013) First report of Impatiens necrotic spot virus on begonia in Bosnia and Herzegovina . Plant Disease , 97 , 1004. [ PubMed ] [ Google Scholar ]
- Vaira, A.M. , Roggero, P. , Luisoni, E. , Masenga, V. , Milne, R.G. and Lisa, V. (1993) Characterization of two tospoviruses in Italy: tomato spotted wilt and impatiens necrotic spot . Plant Pathology , 52 , 530–542. [ Google Scholar ]
- Vasanthi, V.J. , Kandan, A. , Raguchander, T. , Ramanathan, A. , Balasubramanian, P. and Samiyappan, R. (2010) Pseudomonas fluorescens‐based formulations for management of tomato leaf curl gemini virus (TLCV) and enhanced yield in tomato . Archives of Phytopathology , 17 , 553–569. [ Google Scholar ]
- Vasanthi, V.J. , Samiyappan, R. and Vetrivel, T. (2017) Management of tomato spotted wilt virus (TSWV) and its thrips vector in tomato using a new commercial formulation of Pseudomonas fluorescens strain and neem oil . Journal of Entomol Zoology Studies , 5 , 1441–1445. [ Google Scholar ]
- Verhoeven, J. , Roenhorst, J. , Cortes, I. and Peters, D. (1996) Detection of a novel tospovirus in chrysanthemum . Acta horticulture , 432 , 44–51. [ Google Scholar ]
- Verhoeven, T.J. and Roenhorst, J.W. (1998) Occurrence of tospoviruses in the Netherlands . Fourth International Symposium on Tospoviruses and Thrips in Floral and Vegetable Crops pp. 77. Wageningen, The Netherlands: Graduate Schools of Experimental Plant Sciences and Production Ecology.
- Vicchi, V. , Fini, P. and Cardoni, M. (1999) Presence of impatiens necrotic spot tospovirus (INSV) on vegetable crops in Emilia‐Romagna region . Mikologiya I Fitopatologiya , 49 , 52–55. [ Google Scholar ]
- Wang, H. , Lei, Z. , Reitz, S. , Li, Y. and Xu, X. (2013) Production of microsclerotia of the fungal entomopathogen Lecanicillium lecanii (Hypocreales: Cordycipitaceae) as a biological control agent against soil‐dwelling stages of Frankliniella occidentalis (Thysanoptera: Thripidae) . Biocontrol Science and Technology , 23 , 234–238. [ Google Scholar ]
- Webster, C.G. , Frantz, G. , Reitz, S.R. , Funderburk, J.E. , Mellinger, H.C. , McAvoy, E . et al (2015) Emergence of Groundnut ringspot virus and Tomato chlorotic spot virus in vegetables in Florida and the southeastern United States . Phytopathology , 105 , 388–398. [ PubMed ] [ Google Scholar ]
- Webster, C.G. , Perry, K.L. , Lu, X. , Horsman, L. , Frantz, G. , Mellinger, H.C . et al (2010) First report of Groundnut ringspot virus infecting tomato in South Florida . Plant Health Progress . [ Google Scholar ]
- Webster, C.G. , Reitz, S.R. , Perry, K.L. and Adkins, S. (2011) A natural M RNA reassortant arising from two species of plant‐ and insect‐infecting bunyaviruses and comparison of its sequence and biological properties to parental species . Virology , 413 , 216–225. [ PubMed ] [ Google Scholar ]
- Webster, C.G. , Turechek, W.W. , Mellinger, H.C. , Frantz, G , Roe, N. , Yonce, H . et al (2011) Expansion of Groundnut ringspot virus host and geographic ranges in solanaceous vegetables in Peninsular Florida . Plant Health Progress . [ Google Scholar ]
- Wei, M.S. , Li, G.F. , Ma, J. and Kong, J. (2015) First report of Pelargonium flower break virus infecting Pelargonium plants in China . Plant Disease , 99 , 735. [ Google Scholar ]
- Weiss, A. , Dripps, J. and Funderburk, J. (2009) Assessment of implementation and sustainability of integrated pest management programs . Florida Entomologist , 92 , 24–28. [ Google Scholar ]
- Whitfield, A.E. , Campbell, L.R. , Sherwood, J.L. and Ullman, D.E. (2003) Tissue blot immunoassay for detection of Tomato spotted wilt virus in Ranunculus asiaticus and other ornamentals . Plant Disease , 87 , 618–622. [ PubMed ] [ Google Scholar ]
- Whitfield, A.E. , Ullman, D.E. , and German, T.L. (2004) Expression and characterization of a soluble form of tomato spotted wilt virus glycoprotein GN . Journal of Virology , 78 ( 23 ), 13197. [ PMC free article ] [ PubMed ] [ Google Scholar ]
- Whitfield, A.E. , Ullman, D.E. and German, T.L. (2005a) Tospovirus–thrips interactions . Annual Review of Phytopathology , 43 , 459–489. [ PubMed ] [ Google Scholar ]
- Whitfield, A.E. , Ullman, D.E. and German, T.L. (2005b) Tomato spotted wilt virus glycoprotein GC is cleaved at acidic pH . Virus Research , 110 , 183–186. [ PubMed ] [ Google Scholar ]
- Whitfield, A.E. , Falk, B.W. and Rotenberg, D. (2015) Insect vector‐mediated transmission of plant viruses . Virology , 479 , 278–289. [ PubMed ] [ Google Scholar ]
- Wijkamp, I. (1995a) Distinct levels of specificity in thrips transmission of tospoviruses . Phytopathology , 85 , 1069–1074. [ Google Scholar ]
- Wijkamp, I. , Fvande, W. , Goldbach, R. and Peters, D. (2010) Transmission of tomato spotted wilt virus by Frankliniella occidentalis ; median acquisition and inoculation access period . Annual of Applied Biology , 129 , 303–313. [ Google Scholar ]
- Wijkamp, I. (1995b) Virus‐Vector Relationships in the Transmission of Tospoviruses . Ph.D. thesis, Wageningen, The Netherlands.
- Wilson, C.R. , Wilson, A.J. and Pethybridge, S.J. (2000) First report of Tomato spotted wilt virus in common agapanthus . Plant Disease , 84 , 491. [ PubMed ] [ Google Scholar ]
- Wu, K. , Zheng, K.Y. , Zhang, Z.K. , McBeath, J.H. and Dong, J.H. (2016a) First report of Crinum asiaticum as a natural host of tomato zonate spot virus in China . Journal of Plant Pathology , 97 , 76. [ Google Scholar ]
- Wu, S.Y. , Zhang, Z.K. , Gao, Y.L. , Xu, X.N. and Lei, Z.R. (2016b) Interactions between foliage‐ and soil‐dwelling predatory mites and consequences for biological control of Frankliniella occidentalis . BioControl , 61 , 717–727. [ Google Scholar ]
- Wu, S.Y. , Tang, L.D. , Zhang, X.R. , Xing, Z.L. , Lei, Z.R. and Gao, Y.L. (2018) A decade of a thrips invasion in China: lessons learned . Ecotoxicology , 27 , 1032–1038. [ PubMed ] [ Google Scholar ]
- Wu, S.Y. , He, Z. , Wang, E.D. , Xu, X.N. and Lei, Z.R. (2017) Application of Beauveria bassiana and Neoseiulus barkeri for improved control of Frankliniella occidentalis in greenhouse cucumber . Crop Protection , 96 , 83–87. [ Google Scholar ]
- Xiao, L. , Li, Y.Y. , Lan, P.X. , Tan, G.L. , Ding, M. , Li, R.H . et al (2016) First report of Tomato spotted wilt virus infecting cowpea in China . Plant Disease , 100 , 233. [ Google Scholar ]
- Yang, H. , Ozias‐Akins, P. , Culbreath, A.K. , Gorbet, D.W. , Weeks, J.R. , Mandal, B . et al (2004) Field evaluation of Tomato spotted wilt virus resistance in transgenic peanut ( Arachis hypogaea ) . Plant Disease , 88 , 259–264. [ PubMed ] [ Google Scholar ]
- Yoon, J.Y. , Choi, G.S. and Choi, S.K. (2017a) First report of Chrysanthemum stem necrosis virus on Chrysanthemum morifolium in Korea . Plant Disease , 101 , 264. [ Google Scholar ]
- Yoon, J.Y. , Choi, G.S. and Choi, S.K. (2017b) First report of Tomato spotted wilt virus in Eustoma grandiflorum in Korea . Plant Disease , 101 , 515. [ PubMed ] [ Google Scholar ]
- Zarzyńska‐Nowak, A. , Rymelska, N. , Borodynko, N. and Hasiów‐Jaroszewska, B. (2016) The occurrence of Tomato yellow ring virus on tomato in Poland . Plant Disease , 100 , 234. [ Google Scholar ]
- Zhang, X.R. , Lei, Z.R. , Reitz, S.R. , Wu, S.Y. and Gao, Y.L. (2019) Laboratory and greenhouse evaluation of a granular formulation of Beauveria bassiana for control of western flower thrips, Frankliniella occidentalis . Insects , 10 ( 2 ), 58. [ PMC free article ] [ PubMed ] [ Google Scholar ]
- Zhang, Z.J. , Wu, Q.J. , Li, X.F. , Zhang, Y.J. , Xu, B.Y. and Zhu, G.R. (2010) Life history of western flower thrips, Frankliniella occidentalis (Thysan., Thripae), on five different vegetable leaves . Journal of Applied Entomology , 131 , 347–354. [ Google Scholar ]
- Zhang, G.F. , Meng, X.Q. , Min, L. , Qiao, W.N. and Wan, F.H. (2012) Rapid diagnosis of the invasive species, Frankliniella occidentalis (Pergande): a species‐specific COI marker . Journal of Applied Entomology , 136 , 410–420. [ Google Scholar ]
- Zheng, X. , Zhang, J. , Chen, Y. , Dong, J. and Zhang, Z. (2014) Effects of Tomato zonate spot virus infection on the development and reproduction of its vector Frankliniella occidentalis (Thysanoptera: Thripidae) . Florida Entomology , 97 , 549–554. [ Google Scholar ]
- Zheng, Y.X. , Huang, C.H. , Cheng, Y.H. , Kuo, F.Y. and Jan, F.J. (2010) First report of Tomato spotted wilt virus in sweet pepper in Taiwan . Plant Disease , 94 , 920. [ PubMed ] [ Google Scholar ]
- Zindović, J. , Bulajić, A. , Krstić, B. , Ciuffo, M. , Margaria, P. and Turina, M. (2011) First report of Tomato spotted wilt virus on pepper in Montenegro . Plant Disease , 95 , 882. [ PubMed ] [ Google Scholar ]
Thysanoptera in Australia
Frankliniella occidentalis, recognition data, distinguishing features.
Female macroptera. Body variable from yellow to brown, but widespread pest strain usually mainly dark yellow with brown areas medially on each tergite; antennal segments II & VI–VIII brown, III–V yellow with apices variably brown; legs mainly yellow washed with brown; fore wings pale with dark setae. Antennae 8-segmented, III–IV with forked sensorium, VIII longer than VII. Head wider than long; 3 pairs of ocellar setae present, pair III longer than distance between external margins of hind ocelli, arising on anterior margins of ocellar triangle; postocular setae pair I present, pair IV longer than distance between hind ocelli. Pronotum with 5 pairs of major setae; anteromarginal setae slightly shorter than anteroangulars, one pair of minor setae present medially between posteromarginal submedian setae. Metanotum with 2 pairs of setae at anterior margin, campaniform sensilla present. Fore wing with 2 complete rows of veinal setae. Tergites V–VIII with paired lateral ctenidia, ctenidia sometimes weakly developed on IV, on VIII anterolateral to spiracle; posteromarginal comb on VIII complete, with short slender microtrichia arising from triangular bases. Sternites III–VII without discal setae.
Male macroptera. Similar to female but smaller and paler; tergite VIII without marginal comb; IX with median pair of dorsal setae shorter than lateral pair, posterolateral setae stout in larger males; sternites III–VII with transverse pore plate.
Related and similar species
Frankliniella species all have a pair of setae in front of the first ocellus, a complete row of setae on both veins of the forewing, and a pair of ctenidia on tergite VIII situated anterolateral to the spiracles. Most of the 180 described species are known only from the neotropics, but F. schultzei , F. occidentalis and F. williamsi have been widely introduced around the world (Kirk & Terry, 2003). F. occidentalis can usually be recognised by the pale forewings, long postocular setae, presence of metanotal campaniform sensilla, and the rather irregular comb on tergite VIII. However, this species is variable in size and colour, the dark brown form being more common at low temperatures, and the pale yellow form at higher temperatures, but the widespread pest strain is usually more constant in size and colour. Molecular studies have indicated that western flower thrips comprises two species that cannot be distinguished morphologically (Rugman-Jones et al., 2010).
Distribution data
General distribution.
Originally western USA, but now worldwide in temperate areas.
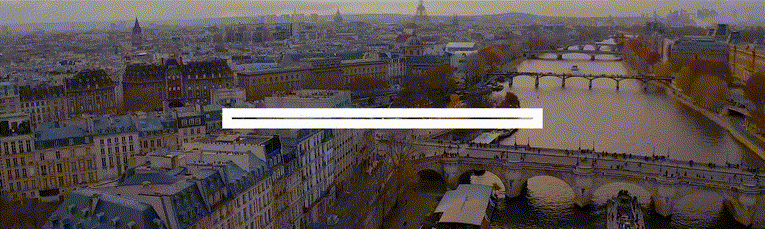
Australian distribution
Locally abundant on cultivated plants across Australia.
Biological data
Life history.
Breeds and feeds on leaves and within flowers. Causes feeding damage on developing fruits.
Host plants
This is a highly polyphagous pest, that is also an important vector of tospoviruses on many crops. However, it also feeds on leaf mites of which it can be a useful biocontrol agent.
Taxonomic data
Current valid name.
Frankliniella occidentalis (Pergande)
Original name and synonyms
- Euthrips occidentalis Pergande, 1895: 392
- Euthrips tritici var . californicus Moulton, 1911: 16
- Euthrips helianthi Moulton, 1911: 40
- Frankliniella tritici var . moultoni Hood, 1914: 38
- Frankliniella nubila Treherne, 1924: 84
- Frankliniella claripennis Morgan, 1925: 142
- Frankliniella canadensis Morgan, 1925: 143
- Frankliniella trehernei Morgan, 1925: 144
- Frankliniella tritici maculata Priesner, 1925: 15
- Frankliniella occidentalis f . brunnescens Priesner,1932: 182
- Frankliniella occidentalis f . dubia Priesner, 1932: 182
- Frankliniella venusta Moulton, 1936: 172
- Frankliniella conspicua Moulton, 1936: 173
- Frankliniella chrysanthemi Kurosawa, 1941: 173
- Frankliniella dahliae Moulton, 1948: 97
- Frankliniella dianthi Moulton, 1948: 98
- Frankliniella syringae Moulton, 1948: 98
- Frankliniella umbrosa Moulton, 1948: 105
Kirk, WDJ & Terry I. 2003. The spread of western flower thrips Frankliniella occidentalis Pergande. Agricultural and Forest Entomology 5 : 301-310.
Hoddle MS, Mound LA & Paris D. 2008. Thrips of California. CD-Rom published by CBIT, Brisbane.
Oz thrips taxa
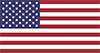
The .gov means it’s official. Federal government websites always use a .gov or .mil domain. Before sharing sensitive information online, make sure you’re on a .gov or .mil site by inspecting your browser’s address (or “location”) bar.
This site is also protected by an SSL (Secure Sockets Layer) certificate that’s been signed by the U.S. government. The https:// means all transmitted data is encrypted — in other words, any information or browsing history that you provide is transmitted securely.
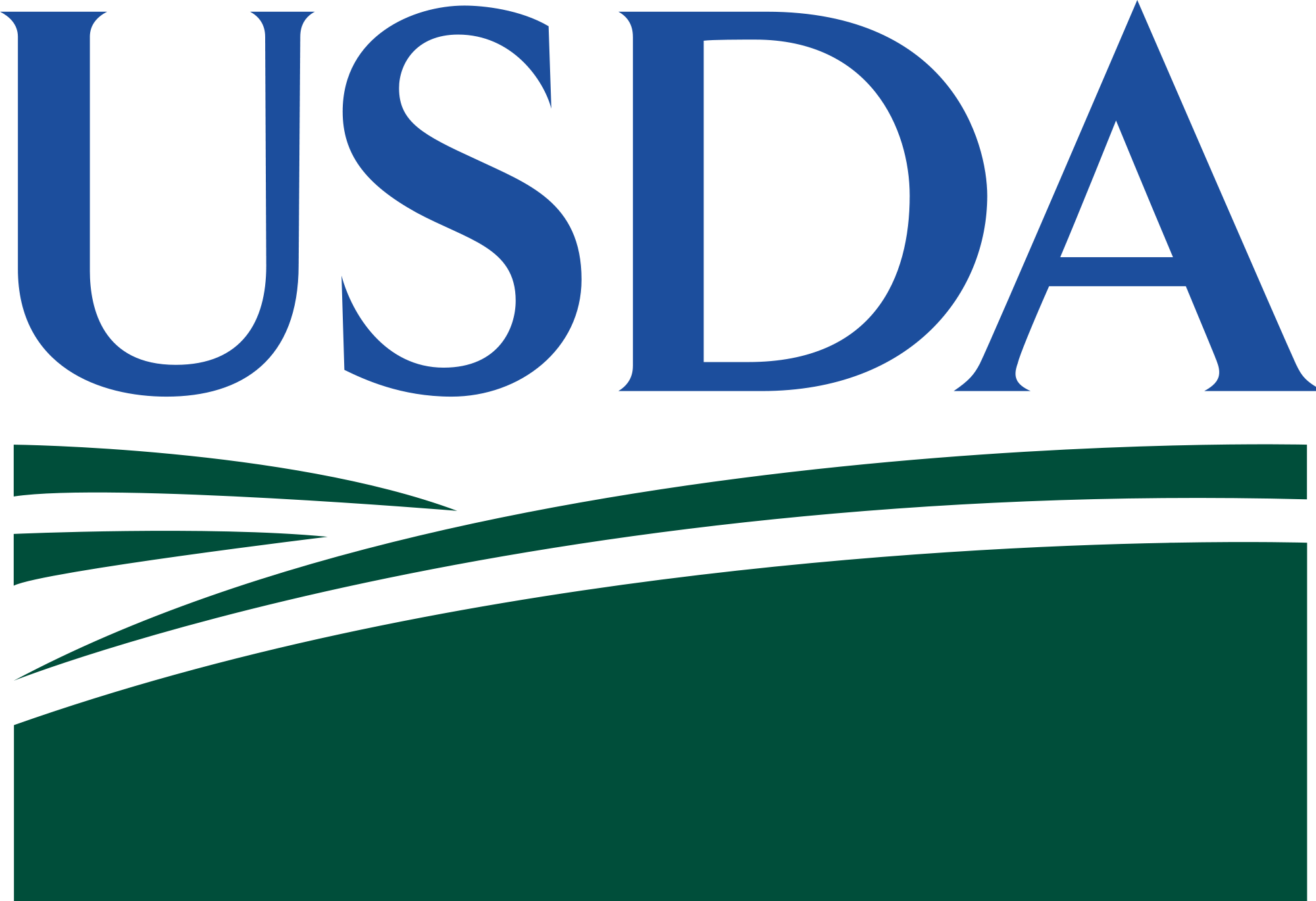
Frankliniella occidentalis
Thank you for visiting nature.com. You are using a browser version with limited support for CSS. To obtain the best experience, we recommend you use a more up to date browser (or turn off compatibility mode in Internet Explorer). In the meantime, to ensure continued support, we are displaying the site without styles and JavaScript.
- View all journals
- My Account Login
- Explore content
- About the journal
- Publish with us
- Sign up for alerts
- Data Descriptor
- Open access
- Published: 08 March 2024
A chromosome-level genome for the flower thrips Frankliniella intonsa
- Wei Song 1 ,
- Jia-Xu Wang 1 , 2 ,
- Li-Jun Cao 1 ,
- Jin-Cui Chen 1 ,
- Wen-Xue Bao 3 ,
- Min Chen 2 &
- Shu-Jun Wei ORCID: orcid.org/0000-0001-7398-0968 1
Scientific Data volume 11 , Article number: 280 ( 2024 ) Cite this article
889 Accesses
1 Altmetric
Metrics details
The flower thrips Frankliniella intonsa (Thysanoptera: Thripidae) is a common insect found in flowers of many plants. Sometimes, F. intonsa causes damage to crops through direct feeding and transmission of plant viruses. Here, we assembled a chromosomal level genome of F. intonsa using the Illumina, Oxford Nanopore (ONT), and Hi-C technologies. The assembled genome had a size of 209.09 Mb, with a contig N50 of 997 bp, scaffold N50 of 13.415 Mb, and BUSCO completeness of 92.5%. The assembled contigs were anchored on 15 chromosomes. A set of 14,109 protein-coding genes were annotated in the genome with a BUSCO completeness of 95.0%. The genome contained 491 non-coding RNA and 0.57% of interspersed repeats. This high-quality genome provides a valuable resource for understanding the ecology, genetics, and evolution of F. intonsa , as well as for controlling thrips pests.
Similar content being viewed by others
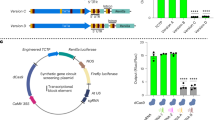
CRISPRi-based circuits to control gene expression in plants
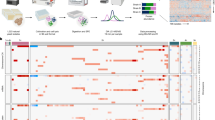
Natural proteome diversity links aneuploidy tolerance to protein turnover
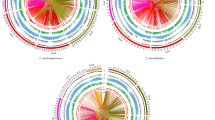
Cicer super-pangenome provides insights into species evolution and agronomic trait loci for crop improvement in chickpea
Background & summary.
Thrips are small insects from the order Thysanoptera. Among the currently described thrips, only about 150 species are recognized as pests 1 . The flower thrips Frankliniella intonsa is a common species found in flowers of many plants. It is native to Eurasia, but now introduced to Oceania and North America 2 , 3 , 4 , 5 , 6 . Despite their small body size allowing for easy dispersal, the distribution of F. intonsa remains limited compared to a cosmopolitan pest from the same genus, the western flower thrips, Frankliniella occidentalis 7 , 8 , 9 . In its native range, F. intonsa was reported as a pest at times 10 but often found alongside other thrips in the field, leading to species competition and displacement 11 , 12 , 13 , 14 , 15 . However, in recent years, F. intonsa has been more frequently treated as a pest of crops 13 , 16 . In some regions, F. intonsa has developed resistance to insecticides used for its control 17 , 18 . In addition, F. intonsa has been found as a vector of plant virus from the genus Tospovirus 19 , 20 , 21 , although its transmission efficacy is lower than F. occidentalis 11 . Therefore, we need to understand its biology, ecology, and evolution, as well as its competition with other species, to reassess the pest status of F. intonsa and develop a proper control strategy 22 , 23 . Well-assembled genomes will provide genetic resources for the study of F. intonsa . Currently, genomes of thrips have been reported for the western flower thrips Frankliniella occidentalis 24 , tobacco thrips Frankliniella fusca 25 , melon thrips Thrips palmi 26 , bean flower thrips Megalurothrips usitatus 27 , 28 and rice thrips Stenchaetothrips biformis 29 . Recently, a parallel study of ours published a genome for F. intonsa that represents the first chromosome-scale genome for the species of the genus Frankliniella 30 . The specimens used for F. intonsa genome sequencing were collected from Zhejiang Province of southern China 30 . Here, we assembled another chromosome-level genome for F. intonsa , which was sequenced from specimens collected from Inner Mongolia of northern China, to enrich the genetic resources of this species. We utilized Illumina short-read sequences to estimate the genome features of F. intonsa . We also employed Oxford Nanopore Technologies (ONT) long-read sequences to assemble a contig-level genome. Furthermore, we utilized chromosome conformation capture (Hi-C) technology to assemble these contigs into a chromosome-level genome.
Sample collection and genomic DNA sequencing
A strain used for genome sequencing was reared for 10 generations in the laboratory at the College of Forestry, Inner Mongolia Agricultural University, Hohhot, China. About 100 unsexed adults collected from Huanghuagou Scenic Area in Chaha’er Right Wing Central Banner, Inner Mongolia, China (E 112°32′03″, N 41°08′17″) were used to establish the strain. Frankliniella intonsa was reared on the seedling of horsebean Vicia faba under the following laboratory conditions: 25 °C, 60% relative humidity and a 16 L:8D photoperiod. The specimens used for sequencing were morphologically identified to avoid the inclusion of other thrips species. About 1,000 adults with pooled male and female samples were utilized for the extraction of high-molecular-weight DNA (HMW DNA) and subsequent library construction. Genomic DNA was extracted from the entire body of pooled individuals using the Qiagen MagAttract HMW DNA Mini Kit, following the manufacturer’s protocol. A short-read DNA library with an insert size of 500 bp was constructed using the Illumina TruSeq DNA PCR-Free HT LPK and sequenced on the Illumina X Ten platforms (Illumina Inc., San Diego, CA, USA). A long-read DNA library with an insert size of 23 kb was prepared according to the manufacturer’s protocol and sequenced using the PromethION model of the ONT platform. The short reads were used for genome survey analysis, including estimating the genome size, and rates of heterozygosity and duplication, as well as for correcting the assembly from the long sequencing reads, while the long reads were used for the contig-level genome assembly. The sequencing process generated 15.55 Gb (73.88X coverage) of clean short-read data and 28.35 Gb (135.65X coverage) of long-read data, respectively (Table 1 ).
Hi-C library construction and sequencing
The chromosome conformation of the genome was captured to determine the order and orientation of the contigs. Approximately 1,000 adults of mixed sex were used for constructing the Hi-C library. The specimens were ground and then cross-linked in a fresh, ice-cold nuclear isolation buffer with a 2% formaldehyde solution for 10 minutes at room temperature. The fixed cells were digested using DpnII (NEB) enzymes and processed according to the standard operating procedure for Hi-C library construction, which included cell lysis, incubation, labelling the DNA ends with biotin-14-dCTP, and performing blunt-end ligation of crosslinked fragments. The Hi-C library was amplified by 12–14 PCR cycles and sequenced on the Illumina NovaSeq. 6000 platform. A total of 26.97 Gb of clean data were generated, representing 120.05X coverage of the genome (Table 1 ).
Genome characteristics estimation
Genome characteristics were estimated based on Illumina short-reads. The raw sequences were trimmed using the software fastp 31 under the default parameters. KMC version 3.0 32 was used to count the K -mer distribution histogram under 17, 21, 27, 31 and 41-mer with parameters ‘-m96 -ci1 -cs10000’ and ‘-cx10000’, based on the trimmed data. The genome size, heterozygosity rate, and duplication rate were estimated using GCE version 2.0 under the default parameters 33 . The estimated genome size decreased as the K -mer increased, ranging from 230 Mb to 255 Mb, similar to a previous study of this species 30 . The genome duplication decreased as the K -mer increased, with values ranging from 2.71% to 3.22%, higher than a previous study of this species (2.04%) 30 . Each K -mer distribution showed double-peaks, indicating a highly complex genome (Table 2 , Fig. 1 ).
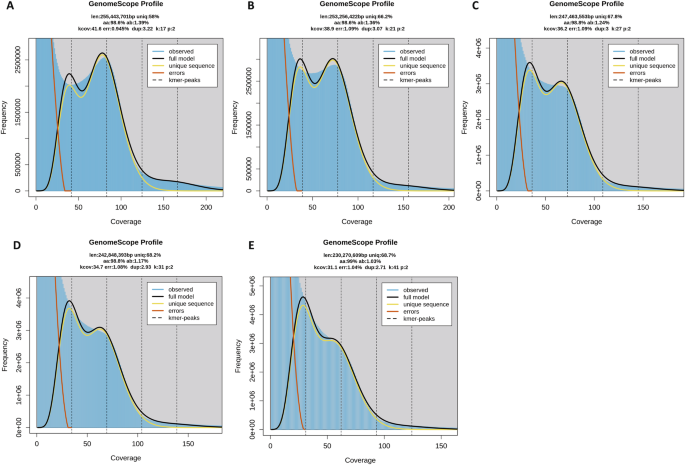
Estimated characteristics of Frankliniella intonsa genome based on Illumina short-read data. Results were obtained in GenomeScope version 2.0 with 17- ( A ), 21- ( B ), 27- ( C ), 31- ( D ) and 41- ( E ) mer. The K -mer distributions showed double peaks: the first peak indicates genome duplication and the highest peak represents a genome size peak. len, estimated genome size in bp; aa, homozygosity rate; ab, heterozygosity rate; dup, duplication rate.
Genome assembly and annotation
The long-reads from ONT were quality-controlled and assembled into contigs using a “correct-then-assemble” strategy in nextDenovo version 2.5.2 34 with parameters ‘read_cutoff = 1k, genome_size = 400 m, pa_correction = 20, sort_options = -m 100 g -t 10, minimap2_options_raw = -t 10, correction_options = -p 15, minimap2_options_cns = -t 10, nextgraph_options = -a 1’. These contigs were then polished three times based on the Illumina short reads using pilon version 1.22 35 under the default parameters. The polished contigs were further assembled into a chromosomal-level genome using Hi-C sequencing data. Low-quality reads and adapters from the Hi-C library were filtered using Trimmomatic version 0.39 36 under the default parameters and then mapped to the assembled contigs using Juicer 37 with default parameters. The reads were grouped into chromosomes using 3D de novo assembly (3D-DNA) version 180922 with parameters ‘–editor_repeat_coverage = 15, -r 2’ 38 . Mistakes were manually adjusted in Juicebox version 2.16.00 ( https://github.com/aidenlab/Juicebox ), and the raw-chromosomes were updated using the script “run-asm-pipeline-post-review.sh” in 3D-DNA again. At last, the repeat-masked high-quality genome assembly was submitted to the online tool Helixer 39 under the invertebrate mode for genome structure annotation. Functional annotation was performed by blasting the proteins against the Uniport/SwissProt database using blastp version 2.12.0+ 40 under the following parameters: ‘-evalue 0.000001 -outfmt 6 -num_threads 128 -num_alignments 1 -seg yes -soft_masking true -lcase_masking -max_hsps 1’. In total 422,839 contigs were assembled into 15 chromosomes (Fig. 2 ). The largest chromosome size was 21.406 Mb and the shortest was 10.106 Mb. We numbered the chromosomes in descending order of their size. The total length of the anchored genome was 209.09 Mb with an N50 of 13.415 Mb. About 57 Mb contigs were not anchored to any chromosome. The anchored genome size is shorter than the estimated genome size and a previously assembled genome for this species 30 . Both anchored and unanchored contigs were submitted to GenBank with accession numbers CM069028.1- CM069042.1. In total, 14,109 protein-coding genes (PCGs) were identified with 9,931 genes have functional annotation 41 . The G + C content of the final genome assembly was 51.75% (Table 2 ).
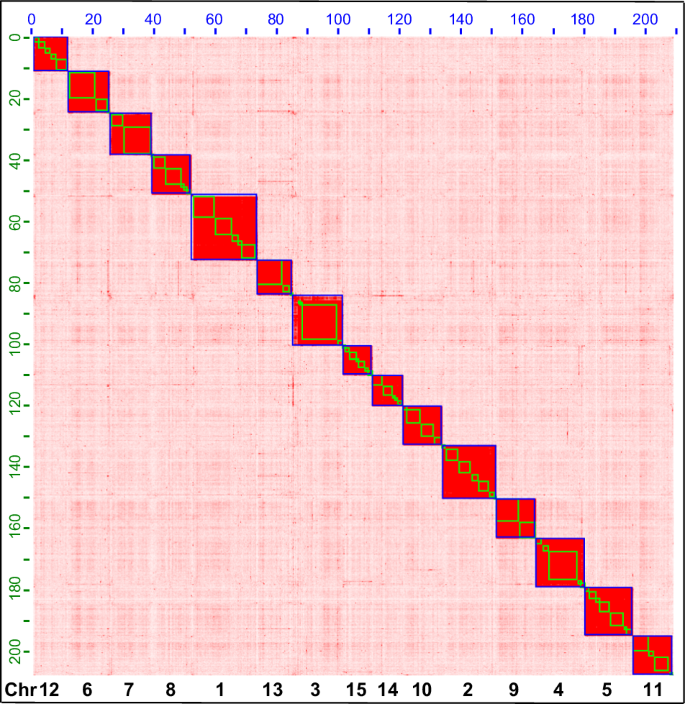
Genome-wide contact matrix of Frankliniella intonsa generated using Hi-C data. Each blue square represents a chromosome, each green square represents a contig. Fifteen chromosomes were anchored under the default parameters of Juicer and 3D-DNA software. Numbers on the top and left axes show the chromosome length in Mb, numbers on the bottom axes show the chromosome number. Chromosomes are numbered based on their size, from the largest to the smallest.
Repeat elements and non-coding RNA predictions
The repetitive elements longer than 1000 bp were identified against the Insecta repeats within RepBase Update (20120418). The identification was performed using RepeatMasker version open-4.0.0 42 (-no_is -norna -xsmall -q) with the search engine RM-BLAST (v2.2.23+). De novo identification of transposable elements (TEs) was performed using RepeatModeler 43 . Non-coding RNAs were identified using Rfam 44 , 45 , while ribosome RNAs (rRNAs) and transfer RNAs (tRNAs) were searched by tRNAscan-SE v2.0 46 and RNAmmer v1.2 47 , both under default parameters. A total of 393,270 transposable elements (TEs) were identified, including 3,903 retroelements with a total length of 452,458 bp, 8,858 DNA transposons and 380,509 Tandem Repeats (TRs) (Table 3 ). We identified 48 miRNAs, 87 snRNAs, 30 snoRNAs, 143 rRNAs and 183 tRNAs in F. intonsa genome (Table 4 ).
Data Records
The genome project was deposited at NCBI under BioProject No. PRJNA1016113. Genomic Illumina sequencing data were deposited in the Sequence Read Archive at NCBI under accession SRR26105494 48 . Genomic ONT sequencing data were deposited in the Sequence Read Archive at NCBI under accession SRP461583 49 . The Hi-C sequencing data were deposited in the Sequence Read Archive at NCBI under accession SRR26122928 50 . The genome assembly, genome annotation, and protein coding genes files were deposited in Figshare under a DOI of https://doi.org/10.6084/m9.figshare.24174591.v5 41 . The final genome assembly was also deposited in GenBank at NCBI under the accession number GCA_035584235.1 51 .
Technical Validation
The extracted high molecular weight (HMW) DNA had an average size of approximately 23 Kb, as determined by pulsed-field gel electrophoresis. To assess the integrity and quality of the genome assembly and the set of protein-coding genes, Benchmarking Universal Single-Copy Orthologs (BUSCO) version 5.4.5 52 was used. For the chromosome-level genome assembly, the BUSCO completeness was 93.3%, 95.6%, 96.1% and 95.0%, based on the Eukaryota, Metazoa, Arthropoda and Insecta (odb_10, released on 2024-01-08) datasets, while the previously assembled genome has a completeness of 96.9%–98.8% 30 . For the protein-coding gene set, the BUSCO completeness was 93.0%, 94.6%, 96.3% and 95.2% based on the Eukaryota, Metazoa, Arthropoda and Insecta datasets, respectively, while the previously assembled genome has a completeness of 89.5%–94.4% 30 . We mapped our Illumina short-read to the assembled genomes using BWA version 0.7.17-r1198-dirty 53 under the BWA-MEM algorithm. The mapping rate of short-reads data to our unmasked chromosomal-level genome and that of Zhang et al . 30 is 81.92% and 87.30%, respectively. We also mapped the Illumina short-read of Zhang et al . 30 and obtained a mapping rate of 84.04% for our genome assembly and 92.80% for the assembly of Zhang et al . 30 .
Code availability
No specific code or script were used in this study.
Mound, L. A., Wang, Z., Lima, E. F. B. & Marullo, R. Problems with the concept of “Pest” among the diversity of pestiferous Thrips. Insects 13 , 61, https://doi.org/10.3390/insects13010061 (2022).
Article PubMed PubMed Central Google Scholar
Zur Strassen, R. Die terebranten thysanopteren Europas und des mittelmeer-gebietes . (Goecke & Evers, 2003).
Nakahara, S. & Foottit, R. G. Frankliniella intonsa (Trybom) (Thysanoptera: Thripidae), an invasive insect in North America. P. Entomol. Soc. Wash . (2007).
Teulon, D. & Nielsen, M. Distribution of western (glasshouse strain) and intonsa flower thrips in New Zealand. New Zealand Plant Protection 58 , 208–212 (2005).
Article Google Scholar
Wang, Z., Mound, L. & Tong, X. Phylogenetic relationships within the Frankliniella genus-group based on morphology, with a revision of Iridothrips (Thysanoptera, Thripidae). Zootaxa 4651 , 141–154–141–154 (2019).
Wang, C. L., Lin, F. C., Chiu, Y. C. & Shih, H. T. Species of Frankliniella Trybom (Thysanoptera: Thripidae) from the Asian-Pacific Area. Zool. Stud. 49 , 824–838 (2010).
Google Scholar
Reitz, S. R. Biology and ecology of the western flower thrips (Thysanoptera: Thripidae): the making of a pest. Fla. Entomol. 92 , 7–13 (2009).
Morse, J. G. & Hoddle, M. S. Invasion biology of thrips. Annu. Rev. Entomol. 51 , 67–89 (2006).
Article CAS PubMed Google Scholar
Reitz, S. R. et al . Invasion biology, ecology, and management of western flower thrips. Annu. Rev. Entomol. 65 , 17–37 (2020).
Kijima, K., Ohno, S., Ganaha-Kikumura, T. & Shimizu, T. Control of flower thrips, Flankliniella intonsa (Trybom) and sweetpotato whitefly, Bemisia tabaci (Gennadius) on sweet pepper in greenhouses in Okinawa, Southwestern Japan, by releasing polyphagous indigenous predator, Campylomma chinensis Schuh (Hemiptera: Miridae). Jpn J. Appl. Entomol. Z. 57 , 167–175, https://doi.org/10.1303/jjaez.2013.167 (2013).
Okuda, S., Okuda, M., Matsuura, S., Okazaki, S. & Iwai, H. Competence of Frankliniella occidentalis and Frankliniella intonsa strains as vectors for Chrysanthemum stem necrosis virus . Eur. J. Plant Pathol. 136 , 355–362 (2013).
Article CAS Google Scholar
Bhuyain, M. M. H. & Lim, U. T. Relative susceptibility to pesticides and environmental conditions of Frankliniella intonsa and F. occidentalis (Thysanoptera: Thripidae), an underlying reason for their asymmetrical occurrence. PloS ONE 15 , e0237876, https://doi.org/10.1371/journal.pone.0237876 (2020).
Article CAS PubMed PubMed Central Google Scholar
Fu, B. et al . Spinetoram resistance drives interspecific competition between Megalurothrips usitatus and Frankliniella intonsa . Pest Manag. Sci. 78 , 2129–2140, https://doi.org/10.1002/ps.6839 (2022).
Pobozniak, M. The occurrence of thrips (Thysanoptera) on food legumes (Fabaceae). J. Plant Dis. Protect. 118 , 185–193, https://doi.org/10.1007/Bf03356402 (2011).
Alim, M. A., Song, J., Seo, H. J. & Choi, J. J. Monitoring thrips species with yellow sticky traps in astringent persimmon orchards in Korea. Appl. Entomol. Zool. 53 , 75–84, https://doi.org/10.1007/s13355-017-0530-z (2018).
Tang, L. D., Guo, L. H., Shen, Z., Chen, Y. M. & Zang, L. S. Comparison of the biology of Frankliniella intonsa and Megalurothrips usitatus on cowpea pods under natural regimes through an age-stage, two-sex life table approach. Bull. Entomol. Res. 113 , 555–564, https://doi.org/10.1017/S0007485323000238 (2023).
Article PubMed Google Scholar
Hiruta, E., Aizawa, M., Nakano, A. & Sonoda, S. Nicotinic acetylcholine receptor α6 subunit mutation (G275V) found in a spinosad-resistant strain of the flower thrips, Frankliniella intonsa (Thysanoptera: Thripidae). J. Pestic. Sci . 43 , D18-007, https://doi.org/10.1584/jpestics.d18-007 PMID - 30479549 (2018).
Gao, Y. F. et al . Geographical and interspecific variation in susceptibility of three common thrips species to the insecticide, spinetoram. J. Pest Sci. 94 , 93–99, https://doi.org/10.1007/s10340-019-01128-2 (2021).
Rotenberg, D., Jacobson, A. L., Schneweis, D. J. & Whitfield, A. E. Thrips transmission of tospoviruses. Curr. Opin. Insect Sci. Virol. 15 , 80–89, https://doi.org/10.1016/j.coviro.2015.08.003 (2015).
Whitfield, A. E., Ullman, D. E. & German, T. L. Tospovirus-thrips interactions. Annu. Rev. Phytopathol. 43 , 459–489 (2005).
Ullman, D. E. et al . Thrips as vectors of tospoviruses. Adv. Bot. Res. 36 , 113–140 (2002).
Liu, X. et al . Weak genetic structure of flower thrips Frankliniella intonsa in China revealed by mitochondrial genomes. Int. J. Biol. Macromol. 231 , 123301, https://doi.org/10.1016/j.ijbiomac.2023.123301 (2023).
Li, H. et al . Chemosensory protein regulates the behavioural response of Frankliniella intonsa and Frankliniella occidentalis to tomato zonate spot virus-Infected pepper ( Capsicum annuum ). PLoS Pathog. 19 , e1011380, https://doi.org/10.1371/journal.ppat.1011380 (2023).
Rotenberg, D. et al . Genome-enabled insights into the biology of thrips as crop pests. BMC Biol. 18 , 1–37 (2020).
Catto, M. A. et al . Pest status, molecular evolution, and epigenetic factors derived from the genome assembly of Frankliniella fusca , a thysanopteran phytovirus vector. BMC Genomics 24 , 1–17 (2023).
Guo, S. K. et al . Chromosome-level assembly of the melon thrips genome yields insights into evolution of a sap-sucking lifestyle and pesticide resistance. Mol. Ecol. Resour. 20 , 1110–1125, https://doi.org/10.1111/1755-0998.13189 (2020).
Ma, L. et al . Chromosome-level genome assembly of bean flower thrips Megalurothrips usitatus (Thysanoptera: Thripidae). Sci. Data 10 , 252 (2023).
Zhang, Z. J. et al . The chromosome-level genome assembly of bean blossom thrips ( Megalurothrips usitatus ) reveals an expansion of protein digestion-related genes in adaption to high-protein host plants. Int. J. Mol. Sci. 24 , 11268, https://doi.org/10.3390/ijms241411268 (2023).
Hu, Q. L., Ye, Z. X., Zhuo, J. C., Li, J. M. & Zhang, C. X. A chromosome-level genome assembly of Stenchaetothrips biformis and comparative genomic analysis highlights distinct host adaptations among thrips. Commun. Biol. 6 , 813, https://doi.org/10.1038/s42003-023-05187-1 (2023).
Zhang, Z. J. et al . Chromosome-level genome assembly of the flower thrips Frankliniella intonsa . Sci. Data 10 , 1–6, https://doi.org/10.1038/s41597-023-02770-3 (2023).
Chen, S. F., Zhou, Y. Q., Chen, Y. R. & Gu, J. fastp: an ultra-fast all-in-one FASTQ preprocessor. Bioinformatics 34 , i884–i890, https://doi.org/10.1093/bioinformatics/bty560 (2018).
Kokot, M., Dlugosz, M. & Deorowicz, S. KMC 3: counting and manipulating k -mer statistics. Bioinformatics 33 , 2759–2761, https://doi.org/10.1093/bioinformatics/btx304 (2017).
Ranallo-Benavidez, T. R., Jaron, K. S. & Schatz, M. C. GenomeScope 2.0 and Smudgeplot for reference-free profiling of polyploid genomes. Nat. Commun. 11 , 1432, https://doi.org/10.1038/s41467-020-14998-3 (2020).
Article CAS PubMed PubMed Central ADS Google Scholar
Hu, J. et al . An efficient error correction and accurate assembly tool for noisy long reads. bioRxiv , 2023.2003. 2009.531669 (2023).
Walker, B. J. et al . Pilon: an integrated tool for comprehensive microbial variant detection and genome assembly improvement. PloS ONE 9 , e112963, https://doi.org/10.1371/journal.pone.0112963 (2014).
Bolger, A. M., Lohse, M. & Usadel, B. Trimmomatic: a flexible trimmer for Illumina sequence data. Bioinformatics 30 , 2114–2120, https://doi.org/10.1093/bioinformatics/btu170 (2014).
Durand, N. C. et al . Juicer provides a one-click system for analyzing loop-resolution Hi-C experiments. Cell Syst. 3 , 95–98 (2016).
Dudchenko et al . De novo assembly of the Aedes aegypti genome using Hi-C yields chromosome-length scaffolds. Science 356 , 92–95 (2017).
Holst, F. et al . Helixer- de novo prediction of primary eukaryotic gene models combining deep learning and a hidden markov model. bioRxiv , https://doi.org/10.1101/2023.02.06.527280 (2023).
Mahram, A. & Herbordt, M. C. NCBI BLASTP on high-performance reconfigurable computing systems. ACM Transactions on Reconfigurable Technology and Systems (TRETS) 7 , 1–20 (2015).
Song, W. & Wei, S. J. Genome assembly and of Frankliniella intonsa . Figshare. https://doi.org/10.6084/m9.figshare.24174591.v5 (2023).
Tarailo-Graovac, M. & Chen, N. S. Using RepeatMasker to identify repetitive elements in genomic sequences. Curr. Protoc. Bioinformatics Chapter 4, 1–14, https://doi.org/10.1002/0471250953.bi0410s25 (2009).
Flynn, J. M. et al . RepeatModeler2 for automated genomic discovery of transposable element families. Proc. Natl. Acad. Sci. USA 117 , 9451–9457 (2020).
Gardner, P. P. et al . Rfam: Wikipedia, clans and the “decimal” release. Nucleic Acids Res. 39 , D141–D145, https://doi.org/10.1093/nar/gkq1129 (2011).
Burge, S. W. et al . Rfam 11.0: 10 years of RNA families. Nucleic Acids Res. 41 , D226–D232, https://doi.org/10.1093/nar/gks1005 (2013).
Schattner, P., Brooks, A. N. & Lowe, T. M. The tRNAscan-SE, snoscan and snoGPS web servers for the detection of tRNAs and snoRNAs. Nucleic Acids Res. 33 , 686–689, https://doi.org/10.1093/nar/gki366 (2005).
Lagesen, K. et al . RNAmmer: consistent and rapid annotation of ribosomal RNA genes. Nucleic Acids Res. 35 , 3100–3108, https://doi.org/10.1093/nar/gkm160 (2007).
NCBI Sequence Read Archive https://identifiers.org/ncbi/insdc.sra:SRR26105494 (2023).
NCBI Sequence Read Archive https://identifiers.org/ncbi/insdc.sra:SRP461583 (2023).
NCBI Sequence Read Archive , (2023). https://identifiers.org/ncbi/insdc.sra:SRR26122928 .
NCBI GenBank https://identifiers.org/ncbi/insdc.gca:GCA_035584235.1 (2024).
Simao, F. A., Waterhouse, R. M., Ioannidis, P., Kriventseva, E. V. & Zdobnov, E. M. BUSCO: assessing genome assembly and annotation completeness with single-copy orthologs. Bioinformatics 31 , 3210–3212, https://doi.org/10.1093/bioinformatics/btv351 (2015).
Li, H. & Durbin, R. Fast and accurate long-read alignment with Burrows-Wheeler transform. Bioinformatics 26 , 589–595, https://doi.org/10.1093/bioinformatics/btp698 (2010).
Download references
Acknowledgements
This work is supported by the National Key R&D Program of China (2023YFD1401200), National Natural Science Foundation of China (31960347), and Programs of Beijing Academy of Agricultural and Forestry Sciences (KJCX20220409, KJCX20240306, JKZX202208).
Author information
Authors and affiliations.
Institute of Plant Protection, Beijing Academy of Agriculture and Forestry Sciences, Beijing, 100097, China
Wei Song, Jia-Xu Wang, Li-Jun Cao, Jin-Cui Chen & Shu-Jun Wei
Beijing Key Laboratory for Forest Pests Control, Beijing Forestry University, Beijing, 100083, China
Jia-Xu Wang & Min Chen
College of Forestry, Inner Mongolia Agricultural University, Hohhot, 010019, China
Wen-Xue Bao
You can also search for this author in PubMed Google Scholar
Contributions
S.J.W. and M.C. conceived the study. J.X.W., W.X.B. and J.C.C. prepared the samples. W.S. and L.J.C. analysed the data. W.S. and S.J.W. wrote and revised the manuscript.
Corresponding author
Correspondence to Shu-Jun Wei .
Ethics declarations
Competing interests.
The authors declare no competing interests.
Additional information
Publisher’s note Springer Nature remains neutral with regard to jurisdictional claims in published maps and institutional affiliations.
Rights and permissions
Open Access This article is licensed under a Creative Commons Attribution 4.0 International License, which permits use, sharing, adaptation, distribution and reproduction in any medium or format, as long as you give appropriate credit to the original author(s) and the source, provide a link to the Creative Commons licence, and indicate if changes were made. The images or other third party material in this article are included in the article’s Creative Commons licence, unless indicated otherwise in a credit line to the material. If material is not included in the article’s Creative Commons licence and your intended use is not permitted by statutory regulation or exceeds the permitted use, you will need to obtain permission directly from the copyright holder. To view a copy of this licence, visit http://creativecommons.org/licenses/by/4.0/ .
Reprints and permissions
About this article
Cite this article.
Song, W., Wang, JX., Cao, LJ. et al. A chromosome-level genome for the flower thrips Frankliniella intonsa . Sci Data 11 , 280 (2024). https://doi.org/10.1038/s41597-024-03113-6
Download citation
Received : 26 September 2023
Accepted : 04 March 2024
Published : 08 March 2024
DOI : https://doi.org/10.1038/s41597-024-03113-6
Share this article
Anyone you share the following link with will be able to read this content:
Sorry, a shareable link is not currently available for this article.
Provided by the Springer Nature SharedIt content-sharing initiative
Quick links
- Explore articles by subject
- Guide to authors
- Editorial policies
Sign up for the Nature Briefing: Translational Research newsletter — top stories in biotechnology, drug discovery and pharma.

- Open access
- Published: 30 December 2019
Controlling the western flower thrips, Frankliniella occidentalis (Pergande) (Thysanoptera: Thripidae) by releasing the predatory phytoseiid mites and pesticides on pepper in a greenhouse
- Ahmad S. Sanad 1 &
- Gamal M. Hassan 2
Egyptian Journal of Biological Pest Control volume 29 , Article number: 95 ( 2019 ) Cite this article
4722 Accesses
2 Citations
Metrics details
Two programs for controlling the western flower thrips (WFT), Frankliniella occidentalis (Pergande) (Thysanoptera: Thripidae), were evaluated. Greenhouse experiments were conducted for reducing F. occidentalis populations on pepper plants. The first program was by releasing four phytoseiid predacious mite species namely Neoseiulus arundonaxi (Metwally and Sanad), N. barkeri (Hughes), N. bicaudus (Wainstein), and Cydnoseius negevi (Swirski and Amitai) in the presence of blue sticky traps. The second one was by applying four sequential applications of different pesticides. Results showed that two releases of N. bicaudus , N. barkeri , and N. arundonaxi in the presence of the blue sticky traps reduced WFT population by 45 to 56%. The best control management of the WFT was by the following insecticides: chlorfenapyr, imidacloprid, azadirachtin, and agricultural potassium soap (third program).
Some abiotic and biotic factors affect pepper production under greenhouse conditions. One of the biotic effects is the western flower thrips (WFT), Frankliniella occidentalis (Pergande) (Thysanoptera: Thripidae). The pest attacks many vegetable plants (Kirişik and Erler 2017 ). F. occidentalis is characterized by its small size, highly reproductive, and spread capability causing a high infestation rate (Cloyd 2009 ). The pest deposits its eggs in plant tissue and pupates in the soil or plant litter surrounding plant on the ground, while nymphs and adults inhabit flowers. In a severe infestation, the appropriate pest management was conducted by multiple applications of insecticides (Bethke et al. 2010 ).
In Egypt, Shalaby ( 2015 ) studied the population fluctuation of F. occidentalis on pepper flowers in a greenhouse, while Hanafy ( 2015 ) studied its host preference and control techniques on cucumber, squash, eggplant, and kidney bean crops. He indicated that acetamiprid and thiamethoxam gave a high reduction in F. occidentalis population on cucumber. Multiuse of synthetic pesticides had led to a resistance against major insecticide groups (Demirozer et al. 2012 ). The potential of F. occidentalis was developed extremely fast resistance against neonicotinoid insecticides (Minakuchi et al. 2013 ). The resistance had been stated for chlorpyrifos and dimethoate (Robb et al. 1995 ) and against permethrin, methomyl, abamectin, bifenthrin, and chlorpyrifos (Immaraju et al. 1992 ). The insecticide residue and toxicity problems on marketable pepper crops, towards beneficial organisms (non-target species) and environmental contamination, generated the need of alternative methods to control WFT. Apart from insecticide control, biocontrol agents such as bugs, Orius spp. (Hemiptera: Anthocoridae) as well as plant extracts, are proved to be suitable and effective methods for F. occidentalis control (Arthurs et al. 2009 and Dogramaci et al. 2011 ).
Several species of the family Phytoseiidae (Acari: Mesostigmata) are important predatory mites on many crops. Numerous phytoseiid species had been reported as effective predators of WFT such as Neoseiulus cucumeris (Oudemans), Amblyseius swirskii (Athias-Henriot), and Amblydromalus limonicus (Garman and McGregor) (Messelink et al. 2006 and Knapp et al. 2013 ). Neoseiulus barkeri (Hughes) was commonly used as a biological control agent for thrips in pepper (Ramakers 1988 ). Moreover, few studies had conducted on this pest on pepper crops in Egypt.
The objective of the present study was to evaluate the reduction of F. occidentalis populations on pepper crop under greenhouse conditions by releasing the predatory phytoseiid mites in combination of using of blue sticky traps and/or by applying pesticides.
Materials and methods
The stored grain mite, Tyrophagous putrescentiae (Schrank) (Acari: Acaridae), was collected from debris samples of wheat bran, using modified Tullgren funnels (Krantz and Walter 2009 ). T. putrescentiae was reared on a mixture of wheat bran and bakery dry yeast at 25 °C in top vented plastic containers (6 × 3 cm, diameters × depth). Mass rearing of the phytoseiid predatory mites was conducted on Tyrophagous putrescentiae . Tested phytoseiid mites were reared constantly on a mixture of all stages of the acarid mite under controlled conditions of 25 ± 2 °C in top-vented plastic containers (6 cm diameter and 10 cm depth). All units were kept in large boxes provided with NaCl-saturated solution for keeping up RH inside the boxes as 75% (Winston and Bates 1960 ). Releasing of phytoseiid mites was conducted by using of a carrier which consisted of a mixture of wheat bran and vermiculate material by 1:1 ratio.
Greenhouse experiments
Two field experiments were conducted in two symmetrical pepper greenhouses (6 m width × 45 m length × 2.5 m height) in a horticulture farm at Qaha district, Qalyobia Governorate, Egypt, during the period from 12 May to 7 July throughout two successive seasons, 2017 and 2018, under natural infestation conditions. The two greenhouses were cultivated by pepper (Top Star var.). Recommended agricultural practices were applied.
The obtained F. occidentalis individuals from the infested greenhouses were preserved in vials containing 70% ethanol alcohol until identification took place at the Taxonomy Research Department, Plant Protection Research Institute (PPRI), Agriculture Research Center (ARC), Giza, Egypt.
The first experiment was carried out to determine the efficiency of four predatory phytoseiid mites (in the presence of blue sticky trap) to reduce F. occidentalis population in pepper greenhouse. The greenhouse was divided into five blocks (9 × 6 m 2 /block and 9 × 2 m 2 /replicates), and each was divided to three replicates. Each block was completely separated by acrylic sheet to prevent mite movement. Thirty blue sticky traps were utilized for attracting of F. occidentalis adults during both seasons in all blocks (each block treatment contained 6 traps). Sticky sheet was (18 cm width × 28 cm length) with a sticky material exposed on both sides. The blue sticky sheet was weekly replaced by new ones. The blue sheet trap (blue sensitive plastic sheet) was obtained from PPRI. The rates and times of releasing of the four predatory phytoseiid mites were presented in Table 1 ; the releasing of four predatory mites was conducted throughout the flowering stage of pepper plant, due to the F. occidentalis infestation level was highly population.
The second experiment was conducted in a neighbor pepper greenhouse, divided as the first one. All management control programs of WFT used spray of chemical, to be compared with untreated block (Table 2 ). Comparison between the four programs of insecticides, against WFT and untreated control (check), was conducted throughout the two successive seasons, 2017 and 2018. Pesticide applications were conducted using calibrated handheld compression sprayer (Kwazar) before application. Each block treatment was completely separated by a plastic sheet to avoid pesticide drift.
The mean numbers of WFT individuals (adults and nymphs/10 pepper flowers) were recorded weekly till the end of the experiments. Also, the mean numbers of WFT adults attracted per blue sheet were recorded. Inspection of WFT per ten pepper flowers was carried out, using a white plan sheet (23 cm width × 33 cm length) as inspection plate. The plate was sprayed by water to avoid insects escaping.
Data analysis
Statistical analyses were performed, using SAS program computer including F -test (SAS Institute 2003 ). Revised least significant differences (LSD) at 5% level of probability were used for comparing means.
Results and discussion
In the untreated check (control), the WFT population recorded three peaks on 19 May, 9 June, and 30 June, 2017, by 33.67, 255.33, and 185.67 individuals per 10 flowers, respectively (Table 3 ). In the treatments, after 2 June 2017 (first date of releasing), the F. occidentalis individuals decreased by releasing the phytoseiid mites. In the treatment of N. barkeri and N. arundonaxi , a low population of WFT (24.33 and 62.67 individuals/10 flowers, respectively, in season 2017) was recorded after 1 week from releasing. At the second releasing of the four tested predatory mites, the population of WFT increased on the block with N. bicaudus and C. negevi (176.67 and 163.73 individuals/10 flowers, respectively, in 2017 season) (Table 3 ).
After the second releasing (30 June 2017), the WFT population was sharply dropped after 2 weeks from releasing all phytoseiid mites. The total mean numbers of WFT were 47.60, 39.86, 67.25, 73.87, and 81.13 individuals per 10 flowers in the case of N. barkeri , N. arundonaxi , N. bicaudus , C. negevi , and untreated check, respectively, during the 2017 season (Table 3 ). Generally, the releasing of both N. barkeri and N. arundonaxi was more effective for WFT suppression in the pepper greenhouse.
In the second season, 2018, the same trend was reported in the four releases of phytoseiid mites against WFT infestation on pepper plants under greenhouse conditions. The releasing of these predators was applied on 19 May and 9 June 2018. During the 2018 season, the population of WFT-infested pepper plant was slowly decreased after the first releasing of predators, while the WFT suppression was rapidly dropped after the two releases in the 2018 season (Table 3 ). Insignificant differences were recorded among the four releases of the phytoseiid mites throughout the season 2018. In general, the block, released by N. barkeri and N. arundonaxi , harbored the low infestation of WFT, F. occidentalis , during the two successive seasons, 2017 and 2018, under greenhouse. A higher reduction percentage rate in population of WFT on pepper was detected when N. barkeri was released in 2017 (74.54, 63.47, and 69.00% reduction after first and second releases and mean reduction after two releases, respectively), followed by N. arundonaxi recording 64.17, 31.36, and 47.77% reduction in the case of first and second releases and overall mean reduction after two releases, respectively (Fig. 1 ). However, N. bicaudus and C. negevi recorded the low reduction percentages (overall means of 40.87 and 36.0% reduction, respectively) on F. occidentalis on pepper plant under greenhouse conditions in the 2017 season (Fig. 1 ).
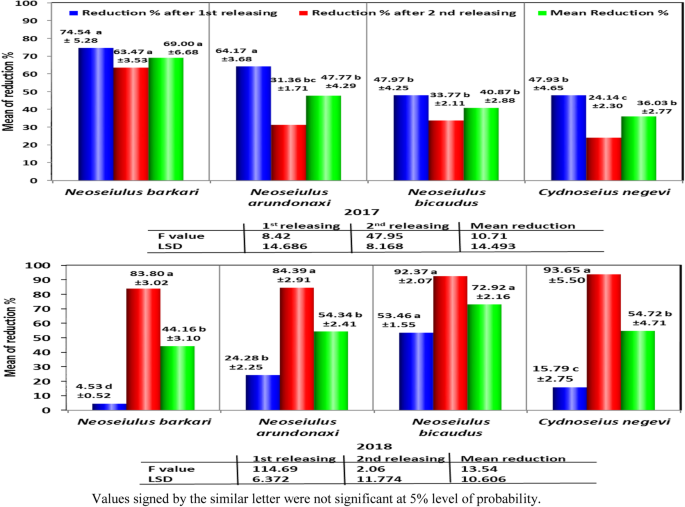
Reduction percentages of Frankliniella occidentalis adults and nymph stages after releasing the phytoseiid mites on pepper greenhouse
On the other hand, N. bicaudus and C. negevi caused the higher reduction of F. occidentalis individuals after two releasing processes than the other two predators, N. barkeri and N. arundonaxi , in season 2018. The reduction percentage was 53.46, 24.28, 15.79, and 4.53% after first releases of N. bicaudus , N. arundonaxi , C. negevi , and N. barkeri , respectively, in the second season 2018. After the second releasing, the reduction was 93.65, 92.37, 84.39, and 83.80% for C. negevi , N. bicaudus , N. arundonaxi , and N. barkeri in 2018, respectively. The overall mean reduction percentage was 72.92, 54.72, 54.34, and 44.16% in the second season 2018 with N. bicaudus , C. negevi , N. arundonaxi , and N. barkeri , respectively (Fig. 1 ).
Generally, the overall mean reduction percentage can be arranged as the following: 56.89, 56.58, 51.05, and 45.38% with N. bicaudus , N. barkeri , N. arundonaxi , and C. negevi , in seasons 2017 and 2018, respectively.
Data in Table 4 show the combined effect of releasing tested phytoseiids and the used of blue sticky trap on pepper plant under greenhouse conditions. The population of WFT individuals/blue sticky sheet recorded three peaks through the tested seasons, 2017 and 2018. The combined effect of predators and sticky traps on the WFT infestations decreased sharply on pepper plants than in the untreated pepper plants (check) (Table 4 ). The use of predators and blue sticky traps together was more effective for thrips suppression. The means of F. occidentalis individuals/blue sheet during 2017 and 2018 seasons are shown in Table 4 . The overall mean of WFT individuals/blue sticky sheet was the highest (63.80 and 34.10 individuals/blue sheet) in block of untreated check, followed by the block of treated one with N. arundonaxi releasing and blue sticky traps (37.90 and 31.20 individuals/blue sticky traps) throughout seasons 2017 and 2018, respectively. Subsequently, the predator-sticky trap combination was more appropriate for WFT controlling on pepper plant under greenhouse conditions through using N. barkeri , N. bicaudus , and N. arundonaxi .
For successful management of F. occidentalis on pepper plants under greenhouse conditions, determination of the thrips damages was critical because the pest had a broad resistance range against insecticides for different chemical categories (Demirozer et al. 2012 and Mouden et al. 2017 ).
In the present study, using of blue sticky traps was more suitable for reducing and attracting of WFT individuals. Broughton and Harrison ( 2012 ) reported that F. occidentalis and T. tabaci were attracted to blue, yellow, and white sticky traps than other tested traps, red, black, and green sticky traps, also in which the blue sticky traps caught the thrip individuals more than the yellow traps. However, current recommendations for attracting of thrips were yellow sticky traps and/or flowers (Hardy et al. 2005 ). Introducing both blue and yellow sticky cards above the plant was the main method for scouting and controlling of thrips by used about 10–40 sticky cards (Cloyd 2009 ).
The obtained data reported that the mean reduction percentage of F. occidentalis infestations could be arranged as 56.89, 56.58, 51.05, and 45.38% with N. bicaudus , N. barkeri , N. arundonaxi , and C. negevi , respectively, during the seasons 2017 and 2018. These results were nearly comparable with those findings by Hessien and Parrella ( 1990 ) who stated that releasing of N. barkeri led to reduce F. occidentalis individual up to 41% and releasing N. cucmeris or N. barkeri was successful for suppressing of thrips in flower crops. The releasing process was conducted per plant as nearly similar to that reported by Hessien and Parrella ( 1990 ) who released N. cucmeris and N. barkeri at (2.5 mites/leaf) against the WFT population. However, the better WFT suppression was achieved when N. cucumeris released at 106 individuals/m 2 / biweekly (Van Driesche et al. 2006 ), while weekly releasing of N. cucumeris (180 individuals/m 2 ) was suitable for F. occidentalis controlling (Bennison et al. 2002 ). For intensification biological control, N. bicaudus , N. barkeri , and N. arundonaxi framework was an augmentative progress against WFT infestation on pepper greenhouse.
Fortunately, pepper greenhouse provides the suitable media for WFT development and infestation. The chemical control of this pest was difficult. According to these programs, the combined of chemical pesticide and bio-pesticide altogether was used in all these programs of WFT control. The mean numbers of F. occidentalis recorded a high value on check pepper block (untreated check) (89.14 individuals/10 flowers), followed by the fourth program (70.35 individuals/10 flowers) during the first season 2017, while the other three programs stated insignificant differences among them during 2017 season (57.21, 54.26, and 45.05 individuals/10 flowers for the first, second, and third programs, respectively). Similarly, the same trend of results was obtained during the second season 2018. The fourth program of pesticides was reported as a high value of WFT individuals/10 flowers, but the low value was observed on each of third, first, and second programs (49.08, 50.63, and 55.95 WFT individuals/10 flowers during season 2018, respectively (Fig. 2 )). Finally, the best WFT control management was the third program, in which sequentially spraying of chlorfenapyr, imidacloprid, azadirachtin, and agricultural potassium soap was used. Data in Fig. 2 illustrates a significant difference between the combination of chemical pesticides and biopesticides in the four tested programs against WFT on pepper plant under greenhouse conditions.

Implement of Frankliniella occidentalis managing by sequentially four insecticide programs in pepper greenhouse
The present data was in harmony with those findings by Hanafy ( 2015 ), in which acetamiprid and thiamethoxam gave a significantly high suppression of F. occidentalis , followed by carbosulfan, spinosad, and spinetoram after 14 days from applications. WFT management key by insecticides can be initiated at the low population of F. occidentalis to avoid overlapping of this pest; once it reaches a high population, it is very difficult to control (Cloyd 2009 ). In the present study, nine different insecticides in four programs were tested against adults and nymphs stages, because the most currently recommended insecticides only suppressed or killed both thrips stages, with no activity on both the eggs and pupal stages (Seaton et al. 1997 ). The primary rule to prevent or reduce the WFT resistance from insecticide was rotating insecticides use (Robb and Parrella 1995 ). In the present study, numerous pesticides were rotated against WFT population on pepper plants under greenhouse conditions. For example, WFT populations were recorded to be resistance against carbamate, pyrethroid, and organophosphates (Kontsedalov et al. 1998 ). Finally, releasing of N. bicaudus and N. barkeri in combination with blue sticky cards reduced the WFT by 56.89 and 56.58%, respectively. The application of the third program (sequential spraying of chlorfenapyr, imidacloprid, azadirachtin, and agricultural potassium soap) gave the best results in reducing the (WFT) (47.1 mean numbers of individuals/10 flowers during the two seasons).
In the present study, releasing of N. bicaudus , N. barkeri , N. arundonaxi , and C. negevi in combination with the blue sticky traps reduced F. occidentalis individuals up to 56% and can be recommended for controlling F. occidentalis on pepper plantations in the greenhouses.
Availability of data and materials
Not applicable
Arthurs S, Mckenzie CL, Chen J, Dogramaci M, Brennan M, Houben K, Osborne L (2009) Evaluation of Neoseiulus cucumeris and Amblyseius swirskii (Acari: Phytoseiidae) as biological control agents of chilli thrips, Scirtothrips dorsalis (Thysanoptera: Thripidae) on pepper. Biol Cont 49(1):91–96
Article Google Scholar
Bennison J, Maulden K, Maher H (2002) Choice of predatory mites for biological control of ground-dwelling stages of western flower thrips within a ‘push-pull’ strategy on pot chrysanthemum. Bulletin OILB/SROP 25:9–12
Google Scholar
Bethke, J.; Chamberlin, J.; Dobbs, J.; Faver, M.; Heinz, K.; Lindquist, R.; Ludwig, S.; Mckenzie, C.L.; Murphy, G.; Oetting, R.; Osborne, L.; Palmer, C.; Parrella, M.; Rechcigl, N. and Yates, R. (2010): Thrips management program for plants for planting. Mid Florida Research & Education Center, Univ. Florida, 1-9 pp.
Broughton S, Harrison J (2012) Evaluation of monitoring methods for thrips and the effect of trap colour and semiochemicals on sticky trap capture of thrips (Thysanoptera) and beneficial insects (Syrphidae, Hemerobiidae) in deciduous fruit trees in Western Australia. Crop Protection 42:156–163
Article CAS Google Scholar
Cloyd RA (2009) Western flower thrips ( Frankliniella occidentalis ) management on ornamental crops grown in greenhouses: have we reached an impasse? Pest Technology 3:1–9
Demirozer O, Tyler-Julian K, Funderburk J, Leppla N, Reitz SR (2012) Frankliniella occidentalis (Pergande) integrated pest management programs for fruiting vegetables in Florida. Pest Manag Sci 68:1537–1545
Dogramaci M, Arthurs SP, Chen J, McKenzie CL, Irrizary F, Osborne LS (2011) Management of Chilli thrips, Scirtothrips dorsalis (Thysanoptera: Thripidae) on peppers by Amblyseius swirskii (Acari: Phytoseiidae) and Orius insidiosus (Hemiptera: Anthocoridae). Biol Cont. 59(3):340–347
Hanafy ARI (2015) Population fluctuation, host preference, damage and control of western flower thrips, Frankliniella occidentalis (Pergande) on some vegetable crops in Egypt. Egypt Acad J Biolog Sci 8(1):145–156
Hardy, S.; Steiner, M.; Thwaite, G. (2005): Plague thrips and western flower thrips. In: Integrated Pest and Disease Management for Australian Summerfruit. NSW Department of Primary Industries, NSW, Summerfruit Australia Inc, 65-69 pp.
Hessein NA, Parrella MP (1990) Predatory mites help control thrips on floriculture crops. California Agriculture 44:19–21
Immaraju JA, Paine TD, Bethke JA, Robb KL, Newman JP (1992) Western flower thrips (Thysanoptera: Thripidae) resistance to insecticides in coastal California greenhouses. J Econ Entomol 85:9–14
Kirişik M, Erler F (2017) The usage possibilities of entomopathogenic fungi in the control of western flower thrips, Frankliniella occidentalis (Pergande) (Thysanoptera: Thripidae). Turkish J Entomol 7(4):293–303
Knapp M, van Houten Y, Hoggerbrugge H, Bolckmans K (2013) Amblydromalus limonicus (Acari: Phytoseiidae) as a biocontrol agent: review and new findings. Acaralogia 53:102–202
Kontsedalov S, Weintraub PG, Horowitz AR, Ishaaya I (1998) Effects of Insecticides on Immature and Adult Western Flower Thrips (Thysanoptera: Thripidae) in Israel. J Econ Entomol 91(5):1067–1071
Krantz GW, Walter DE (2009) A manual of acarology: third edition, Texas Tech. University Press, USA, 816 pp
Messelink GJ, Steenpaal SEF, Ramakers PMJ (2006) Evaluation of phytoseiid predators for control of Western flower thrips on greenhouse cucumber. Biocontrol 51:753–768
Minakuchi C, Inano Y, Shi X, Song D, Zhang Y, Miura K, Sonoda S (2013) Neonicotinoid resistance and cDNA sequences of nicotinic acetylcholine receptor subunits of the western flower thrips Frankliniella occidentalis (Thysanoptera: Thripidae). Appl Entomol Zool 48(4):507–513
Mouden S, Sarmiento KF, Klinkhamer PGL, Leiss KA (2017) Integrated pest management in western flower thrips: past, present and future. Pest Manag Sci 73:813–822
Ramakers PMJ (1988) Population dynamics of the thrips predators Amblyseius mckenziei and Amblyseius cucumeris (Acarina: Phytoseiidae) on sweet pepper. Netherlands J Agricul Sci 36:247–252
Robb KL, Newman J, Virzi JK, Parrella MP (1995) Insecticide resistance in western flower thrips. In: Parker BL, Skinner M, Lewis T (eds) Thrips Biology and Management. Plenum Press, New York, pp 341–346
Chapter Google Scholar
Robb KL, Parrella MP (1995) IPM of western flower thrips. In: Parker BL, Skinner M, Lewis T (eds) Thrips Biology and Management. Plenum Press, New York, pp 365–370
SAS Institute (2003): Statistical Analysis System, SAS Release 9.1 for windows, SAS Institute Inc.Cary, NC, USA.
Seaton KA, Cook DF, Hardie DC (1997) The effectiveness of a range of insecticides against western flower thrips ( Frankliniella occidentalis ) (Thysanoptera:Thripidae) on cut flowers. Aust J Agric Res 48:781–788
Shalaby, H.H. (2015): Preliminary study on the control of western flower thrips, Frankliniella occidentalis (Pergande) in pepper crop greenhouses in Qalyubia Governorate, Egypt. J Plant Prot Path., Mansoura Univ., 6(1): 155-167.
Van Driesche RG, Lyon S, Stanek EJ, Xu B, Nunn C (2006) Evaluation of efficacy of Neoseiulus cucumeris for control of Western flower thrips in spring bedding crops. Biol Control 36:203–215
Winston PW, Bates DH (1960) Saturated solutions for the control of humidity in biological research. Ecology 41:232–237
Download references
Acknowledgements
Author information, authors and affiliations.
Vegetable and Aromatic plant Mites Department, Plant Protection Research Institute, Agricultural Research Center, 12618 Dokii, Giza, Egypt
Ahmad S. Sanad
Vegetable, Medicinal, Aromatic and Ornamental Plant Pests Department, Plant Protection Research Institute, Agricultural Research Center, Giza, Egypt
Gamal M. Hassan
You can also search for this author in PubMed Google Scholar
Contributions
ASS and GMH contributed to the suggesting and putting of the idea, preparing of the manuscript writing and finishing of the paper, and data analysis. All authors contribute by 100% participation. Both authors read and approved the final manuscript.
Corresponding author
Correspondence to Gamal M. Hassan .
Ethics declarations
Ethics approval and consent to participate, consent for publication, competing interests.
The authors declare that they have no competing interests.
Additional information
Publisher’s note.
Springer Nature remains neutral with regard to jurisdictional claims in published maps and institutional affiliations.
Rights and permissions
Open Access This article is distributed under the terms of the Creative Commons Attribution 4.0 International License ( http://creativecommons.org/licenses/by/4.0/ ), which permits unrestricted use, distribution, and reproduction in any medium, provided you give appropriate credit to the original author(s) and the source, provide a link to the Creative Commons license, and indicate if changes were made.
Reprints and permissions
About this article
Cite this article.
Sanad, A.S., Hassan, G.M. Controlling the western flower thrips, Frankliniella occidentalis (Pergande) (Thysanoptera: Thripidae) by releasing the predatory phytoseiid mites and pesticides on pepper in a greenhouse. Egypt J Biol Pest Control 29 , 95 (2019). https://doi.org/10.1186/s41938-019-0186-9
Download citation
Received : 04 August 2019
Accepted : 29 October 2019
Published : 30 December 2019
DOI : https://doi.org/10.1186/s41938-019-0186-9
Share this article
Anyone you share the following link with will be able to read this content:
Sorry, a shareable link is not currently available for this article.
Provided by the Springer Nature SharedIt content-sharing initiative
- Frankliniella occidentalis
- Predatory phytoseiid mites
- Insecticides
- Blue sticky trap
- Biological control
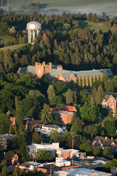
Virtual Tour
Experience University of Idaho with a virtual tour. Explore now
- Discover a Career
- Find a Major
- Experience U of I Life
More Resources
- Admitted Students
- International Students
Take Action
- Find Financial Aid
- View Deadlines
- Find Your Rep
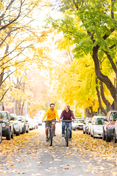
Helping to ensure U of I is a safe and engaging place for students to learn and be successful. Read about Title IX.
Get Involved
- Clubs & Volunteer Opportunities
- Recreation and Wellbeing
- Student Government
- Student Sustainability Cooperative
- Academic Assistance
- Safety & Security
- Career Services
- Health & Wellness Services
- Register for Classes
- Dates & Deadlines
- Financial Aid
- Sustainable Solutions
- U of I Library
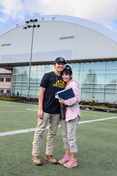
- Upcoming Events
Review the events calendar.
Stay Connected
- Vandal Family Newsletter
- Here We Have Idaho Magazine
- Living on Campus
- Campus Safety
- About Moscow
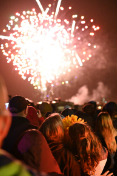
The largest Vandal Family reunion of the year. Check dates.
Benefits and Services
- Vandal Voyagers Program
- Vandal License Plate
- Submit Class Notes
- Make a Gift
- View Events
- Alumni Chapters
- University Magazine
- Alumni Newsletter
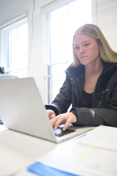
U of I's web-based retention and advising tool provides an efficient way to guide and support students on their road to graduation. Login to VandalStar.
Common Tools
- Administrative Procedures Manual (APM)
- Class Schedule
- OIT Tech Support
- Academic Dates & Deadlines
- U of I Retirees Association
- Faculty Senate
- Staff Council
Integrated Pest Management Center
University of Idaho Extension
Web: uidaho.edu/extension/ipm
Pest Common Name
- Western Flower Thrips ( Frankliniella occidentalis )
- Onion Thrips ( Thrips tabaci )
Host plants
- Crops: alfalfa, barley, clover, corn, onions, potato, wheat and others
- Vegetables: beans, brassicas, corn, cucurbits, garlic, leafy greens, onions, peas, peppers, tomatoes and others
- Fruits: apple, nectarine, peach, grapes, others
- Many ornamentals, grasses and weeds
- Greenhouse-grown plants
Western flower thrips and onion thrips are the primary damaging thrips species in Idaho. Adult thrips have thin, elongated bodies, feather-like wings and small, dark eyes (Figure 1). Thrips range in color from brown to yellow to cream, depending on the species, sex and life stage. Immature thrips (larvae) are also elongated, and mostly resemble adults though smaller and often more translucent (Figure 2). Thrips eggs are only visible with a microscope but scarring on some plant tissues, particularly young fruitlets, may be visible with the naked eye.
Differentiating between species of thrips can be key in diagnosing problems and managing infestations. In general, adult western flower thrips are generally around 1/32-1/16 inch (0.8-1.5 mm) in length, while onion thrips are slightly smaller (Figure 3). Western flower thrips have more hair on the front of their thorax than onion thrips, a chevron pattern on their abdominal segments where onion thrips have straight bands, and their antennae are segmented into eight segments as opposed to the onion thrips 7 (Figure 3). Keep in mind that differentiation between these thrips species often requires a microscope or consulting an entomologist or extension agent.

Western flower thrips and onion thrips both lay their eggs within plant tissues. Larvae hatch from eggs four to 15 days after eggs are laid and feed on plant tissues for two to three weeks before dropping into the soil to pupate. Adults can remain active, feeding and mating, for about a month. A single female can produce up to 200 eggs in her lifetime. Adults and larvae appear to prefer to feed on pollen, and so can often be found within blossoms. Thrips are poor fliers, but in windy conditions they can disperse great distances.
Thrips can damage plants in multiple ways. First, oviposition (egg laying) within plant tissues can leave scarring. This can be particularly problematic in fruits such as apples and nectarine, and some vegetables such as bean and pea pods (Figure 4).
The feeding of thrips can leave brown or silvery scarring and stippling on shoots, stems and leaves, and can also damage flowers. Feeding of thrips usually only causes cosmetic damage, though heavier infestations can cause leaf curling, stunted growth and death of the plant. Furthermore, hot and dry conditions can favor outbreaks of thrips while plants are already stressed, leading to more severe damage.
Lastly, thrips act as a carrier and transmitter of important plant viruses, which can spread through plant populations and cause much more severe damage and widespread plant death. Western flower thrips and onion thrips vector some key viruses such as tomato spotted wilt virus, tomato chlorotic spot virus and iris yellow spot virus. The symptoms of each virus vary between plant species and under different environmental conditions, so consult a specialist for their identification.
Adult thrips become active in the spring and are most easily monitored for using sticky traps, visual scouting, or by looking for characteristic oviposition (Figure 4) or feeding damage (silver or bleached surface scarring, dark fecal spots (Figure 5). Often it is best to focus visual scouting efforts on plant blooms. Monitoring thrips is difficult because of their size. Thrips are best identified under a loupe or low powered microscope or taken to your local Extension educator.
Monitoring practices and economic thresholds differ between crops.
In vegetable crops — To monitor thrips, shake leaves over a white paper and look for thrips to fall off onto the paper. Also look at the bases of leaves and new growth where thrips sometimes congregate (Figure 5). Though no formal economic threshold exists for most vegetable crops, if more than 30 thrips are observed per leaf and damage is being seen on the plants, control may be merited in high value crops. If thrips infestations are severe when plants are not fully established (until three to four weeks after planting) treatment may be warranted.

In onions — Monitor for thrips in onions by looking for the characteristic sliver damage they cause, and by scouting for congregations of thrips within the necks of plants (Figure 6). Scout for thrips in multiple, random places around the field. Keep in mind that young plants, and plants in the early bulbing stage, are most likely to suffer economic damage from thrips, so scouting should be more frequent leading up to and through this stage. There is no formal economic threshold for thrips in onions in Idaho, but there are some general thresholds that have helped guide economic management in Idaho and neighboring states.
- For young plants in the early season, consider treatment when an average of two or more thrips are found per plant
- For plants in the mid- season, consider treatment when an average of 20 or more thrips are found per plant
- More mature plants, in the late season, can tolerate larger numbers of thrips without suffering economic damage
- Onions to be sold in the fresh market with leaves attached suffer severely reduced marketability when thrips scarring is present, so consider treatment as soon as thrips feeding damage is detected

In fruit trees — Monitor blossoms for thrips by shaking them vigorously over a white cup or piece of paper, and count only the large, adult female thrips that fall out. This can be done without removing blossoms from the tree. Sample four blossom clusters per tree and at least four trees per block and cultivar.
In hay crops such as alfalfa — Monitor thrips by inserting leaves from multiple stems into a jar with rubbing alcohol and let sit for several hours. Strain the alcohol through a coffee filter and count the number of thrips captured. Control is merited when more than 50 thrips are present for every six stems.
Primary Management Tactics
Sanitation is a key practice in the management of thrips and the viruses they vector. Remove all unneeded plant material promptly after harvest or at the end of the season and manage weeds that can serve as reservoirs both for thrips and viruses. Thrips damage rarely reaches economic levels, so take note of economic thresholds when considering treatment options.
- Prune infected leaves and stems to reduce population sizes
- In greenhouse settings, use a thrips screen to cover all openings to avoid colonization
- In greenhouses and gardens, younger herbaceous plants can be protected by a thrips screen row cover similar to a low tunnel, which can then be removed when the plant is more mature and can handle more thrips damage
- Plant thrips resistant cultivars
- Avoid planting susceptible plants near weedy areas or next to fields or grasslands
- Control weeds close to susceptible plants
- Consider the use of reflective mulches which interfere with thrips ability to locate host plants
- Avoid the use of broad-spectrum insecticides where possible to conserve resident predators of thrips such as lacewings, minute pirate bugs, ladybugs and predatory thrips
- There is a wide variety of insecticides labeled for thrips, though they have rapidly developed tolerance to many. To reduce the risk of tolerance, alternate insecticides from different groups if multiple applications are necessary.
- Applications should be made only when abundance or damage reaches economic levels, as many insecticides that are labeled for thrips are also toxic to beneficial insects
- Thrips are present throughout the year and become active in the spring. Applications in the spring may help keep populations from increasing.
- Insecticidal soaps and neem oil may be effective at controlling thrips, while reducing risk to beneficials and the environment compared to many synthetic insecticides
- Recommendations for pesticides to use in the management of thrips in various crops and landscape plants can be found on the PNW Pest Management Handbooks website
Pesticide Warning Always read and follow the instructions printed on the pesticide label. The pesticide recommendations in this University of Idaho webpage do not substitute for instructions on the label. Pesticide laws and labels change frequently and may have changed since this publication was written. Some pesticides may have been withdrawn or had certain uses prohibited. Use pesticides with care. Do not use a pesticide unless the specific plant, animal or other application site is specifically listed on the label. Store pesticides in their original containers and keep them out of the reach of children, pets and livestock. Trade Names — To simplify information, trade names have been used. No endorsement of named products is intended nor is criticism implied of similar products not mentioned. Groundwater — To protect groundwater, when there is a choice of pesticides, the applicator should use the product least likely to leach.
Photo credit
- Figure 1. Jack T. Reed, Mississippi State University, Bugwood.org
- Figure 2. Erik J. Wenninger, University of Idaho
- Figure 3. Alton N. Sparks, Jr., University of Georgia, Bugwood.org
- Figures 4-6. Whitney Cranshaw, Colorado State University, Bugwood.org
Joseph Sagers , Extension Educator Desiree Wickwar , Entomologist, IPM Project Manager 2023
New Data on the Thrips (Thysanoptera) Fauna of Yakutia
- Published: 15 April 2024
- Volume 103 , pages 990–993, ( 2023 )
Cite this article
- T. G. Evdokarova 1 &
- E. A. Afanasieva 1
18 Accesses
Explore all metrics
New data are presented on two invasive species of thrips in Yakutia: Frankliniella lilivora Kurosawa, 1937, new to the fauna of Russia, and Liothrips vaneeckei Priesner, 1920, new to the fauna of Yakutia. Morphological description of F. lilivora , specific to lily bulbs, and a key to the Russian Frankliniella species are provided.
This is a preview of subscription content, log in via an institution to check access.
Access this article
Price includes VAT (Russian Federation)
Instant access to the full article PDF.
Rent this article via DeepDyve
Institutional subscriptions
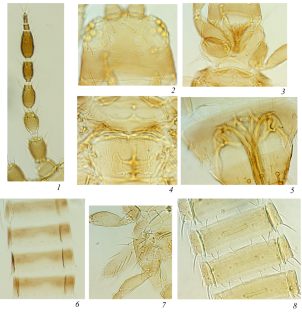
Derbeneva, N.N., Thrips (Thysanoptera) of Crimea, Entomol. Obozr ., 1974, vol. 53, no. 3, p. 602.
Google Scholar
Desyatkin, R.V., Pochvoobrazovanie v termokarstovykh kotlovinakh—alasakh kriolitozony (Soil Formation in Thermokarst Depressions—Alases of Cryolithozone), Novosibirsk: Nauka, 2008.
Dyadechko, N.P., Tripsy, ili bakhromchatokrylye (Thysanoptera) nasekomye evropeiskoi chasti SSSR (Thrips, or Physopod Insects (Thysanoptera) of the European Part of the USSR), Kiev, 1964.
Evdokarova, T.G., Invasive thrips in Central Yakutia, Zashch. Karant. Rast. , 2020, vol. 8, p. 33.
Evdokarova, T.G. and Velikan’, V.S., Material on the fauna of thrips (Insecta, Thysanoptera) Central Yakutia, Entomol. Obozr ., 2011, vol. 90, no. 1, p. 118.
John, O., Ein neuer Haplothrips aus Fergana nebst Verzeichnis der bisher in Rußland gefundenen Thysanopteren, Entomol. Mitt. , 1925, vol. 15, no. 1, p. 17.
Kurosawa, M., Descriptions of four new thrips in Japan, Kontyû , 1937, vol. 11, no. 3, p. 266.
Masumoto, M. and Okajima, S., Occurrence in Japan of Frankliniella hemerocallis (Thysanoptera, Thripidae), with description of larva and key to Frankliniella species from Japan, Zootaxa , 2013, vol. 3718, no. 1, p. 58.
Meshcheryakov, A.A., Order Thysanoptera, or thrips, in Opredelitel’ nasekomykh Dal’nego Vostoka SSSR (Key to the Insects of the Far East of the USSR), Lehr., P.A., Ed., Leningrad: Nauka, 1986, pp. 380–431.
Priesner, H., Moor-Thripse, Konowia , 1922, vol. 1, p. 177.
Scalon, O., Sur les Thysanoptères du Bassin de la riviere Abacan, Ann. Soc. Entomol. Belg ., 1935, vol. 75, p. 35.
Schliephake, G., Ein Beitrag zum Vorkommen der Thysanopteren im Kursker Gebiet (Sowjetunion), Wissenschaftliche Hefte der Pädagogischen Hochschule “W. Ratke” Köthen , 1977, vol. 4, no. 12, p. 124.
Sorokopudova, O.A., Lilii v kul’ture (Lilies in Culture), Moscow: All-Russia Selection Technology Institute of Horticulture and Nursery; Saratov: Amirit, 2019.
ThripsWiki, ThripsWiki—Providing Information on the World’s Thrips , 2023 (accessed 01.03.2023). http://thrips.info/wiki/
Vierbergen, G., Thysanoptera. Fauna Europaea version 2017.06 , 2023 (accessed 01.03.2023). https://fauna-eu.org
Wang, Z., Mound, L., and Tong, X., Frankliniella species from China, with nomenclatural changes and illustrated key (Thysanoptera, Thripidae), ZooKeys , 2019, vol. 873, p. 43. https//doi.org: 10.3897/zookeys.873.36863
Download references
ACKNOWLEDGMENTS
The authors express gratitude to N.N. Vinokurov (IBPC, Siberian Branch, Russian Academy of Sciences), B. Vierbergen (Netherlands Institute for Vectors, Invasive Plants and Plant Health), and M. Ulitzka (Offenburg, Germany) for valuable advice during the work on the paper, help in identification of the species, and for the literature supplied, and also to A. Hastenpflug-Vesmanis (Senckenberg Museum, Frankfurt am Main) for granting copies of the necessary publications.
The research was carried out within the state assignment of Ministry of Science and Higher Education of the Russian Federation (theme no. 0297-2021-0044, reg. no.121020500194-9).
Author information
Authors and affiliations.
Institute for Biological Problems of Cryolithozone, Siberian Branch, Russian Academy of Sciences, 677980, Yakutsk, Russia
T. G. Evdokarova & E. A. Afanasieva
You can also search for this author in PubMed Google Scholar
Corresponding author
Correspondence to T. G. Evdokarova .
Ethics declarations
Statement on the welfare of animals . All applicable international, national, and institutional guidelines for the care and use of animals were followed. All procedures performed in studies involving animals were in accordance with the ethical standards of the institution or practice at which the studies were conducted. The authors declare that they have no conflict of interest.
Additional information
Publisher's Note . Pleiades Publishing remains neutral with regard to jurisdictional claims in published maps and institutional affiliations.
Rights and permissions
Reprints and permissions
About this article
Evdokarova, T.G., Afanasieva, E.A. New Data on the Thrips (Thysanoptera) Fauna of Yakutia. Entmol. Rev. 103 , 990–993 (2023). https://doi.org/10.1134/S0013873823090063
Download citation
Received : 15 November 2022
Revised : 01 December 2023
Accepted : 01 December 2023
Published : 15 April 2024
Issue Date : December 2023
DOI : https://doi.org/10.1134/S0013873823090063
Share this article
Anyone you share the following link with will be able to read this content:
Sorry, a shareable link is not currently available for this article.
Provided by the Springer Nature SharedIt content-sharing initiative
- Frankliniella lilivora
- Liothrips vaneeckei
- Central Yakutia
- Find a journal
- Publish with us
- Track your research
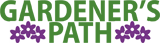
How to Identify and Manage Fuchsia Gall Mites
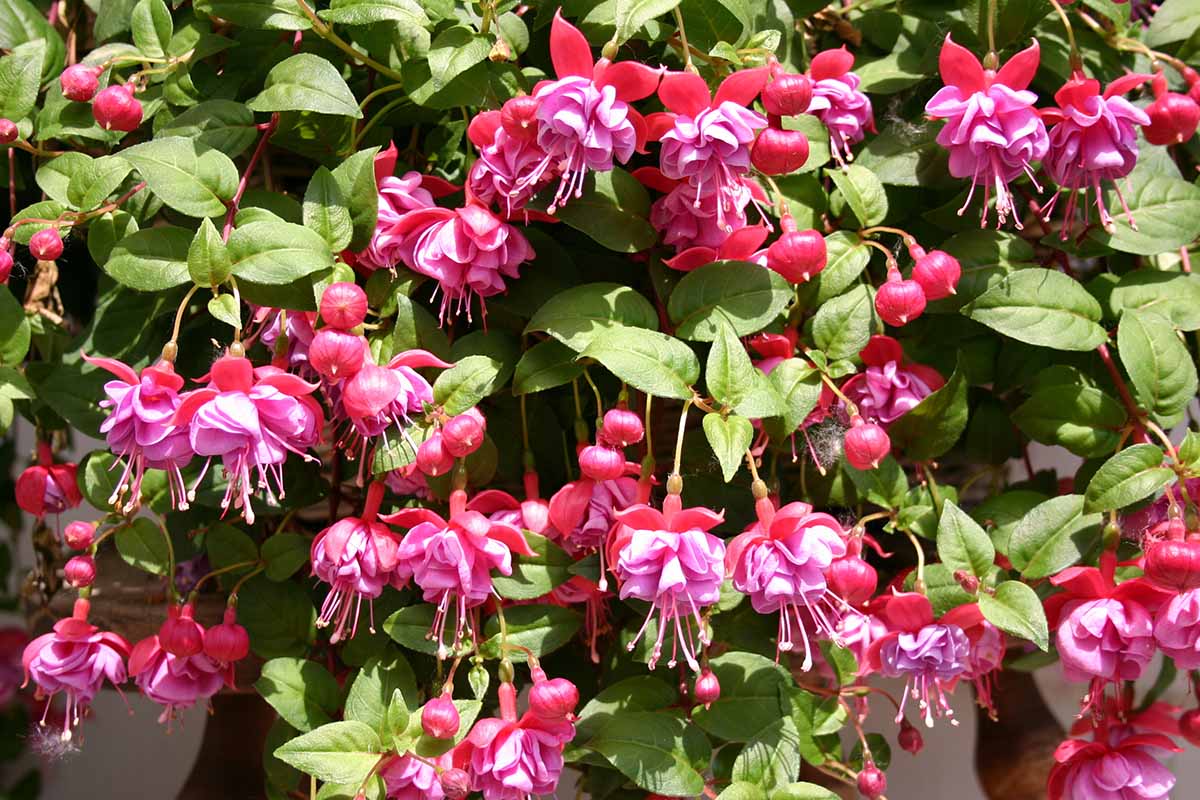
Who knew a microscopic pest could do so much damage? I guess anyone who has ever had a serious case of the flu understands how tiny pathogens can cause problems, but that doesn’t make it any less frustrating.
As gardeners, we often expect plant pests to be obvious, but fuchsia gall mites are invisible to the naked eye. You’d need a microscope to see them.
However, the damage they do is far from small. These pests can take your fuchsia from a big, lush, floriferous beauty to a funky, scraggly mess in no time flat.
We link to vendors to help you find relevant products. If you buy from one of our links, we may earn a commission .
If they’re invisible, how do you even know your fuchsia has an infestation? And what can you do about it if you confirm their presence? That’s what this guide aims to explain.
We’ll help you understand the nature of this pest, how to identify the symptoms they cause, and what to do about it. Here’s what you can expect:
What You’ll Learn
About gall mites.
Alas, identifying these pests isn’t going to be totally straightforward. To give you a leg up, let’s learn a bit about these tiny troublemakers.
Fuchsia gall mites ( Aculops fuchsiae ) are microscopic eriophyid mites that draw the sap out of plants using their sucking mouthparts.
The good news is they only attack species in the Fuchsia genus. The bad news is they attack all species in the genus.
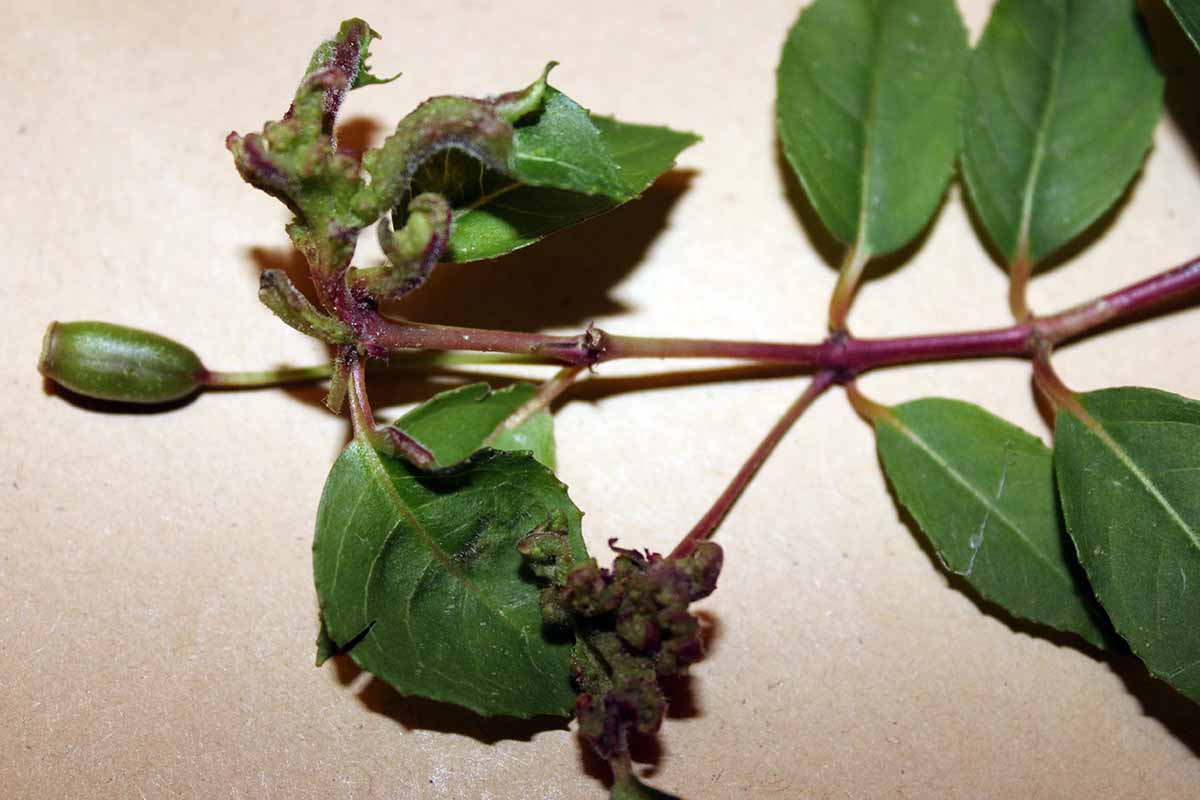
You can’t see them with the naked eye because the adults are just 0.20 to 0.25 millimeters long. You’d need a 40x strength microscope to be able to make them out.
These mites have four life stages: egg, larvae, nymph, and adult.
Once temperatures reach about 64°F and remain there for several days, the eggs hatch about a week after being laid, and the entire life cycle is completed in about 21 days.
That means there is lots of time for populations to build up during the warm months. Then, all stages can overwinter in the plant, waiting for their chance to burst forth again the following spring.
The pests aren’t typically found in greenhouses , as they generally prefer cool temperatures.
But conversely, they don’t survive in low temperatures, either. In other words, fuchsia gall mites do best in the same sort of environment that fuchsias prefer.
The mites can travel on wind or hitch a ride on hummingbirds and bees as they visit the flowers. Gardeners spread them on contaminated tools or clothing.
When they find a host, the adults move into the plant’s hairs and the folds of existing galls to live, feed, and reproduce.
These pests were first identified in 1971 in Brazil where lots of Fuchsia species grow indigenously.
They reached North America and were discovered in San Francisco county in 1983, completely decimating the fuchsia industry in the state, spreading quickly along the cool coast and more slowly into the warmer inland areas.
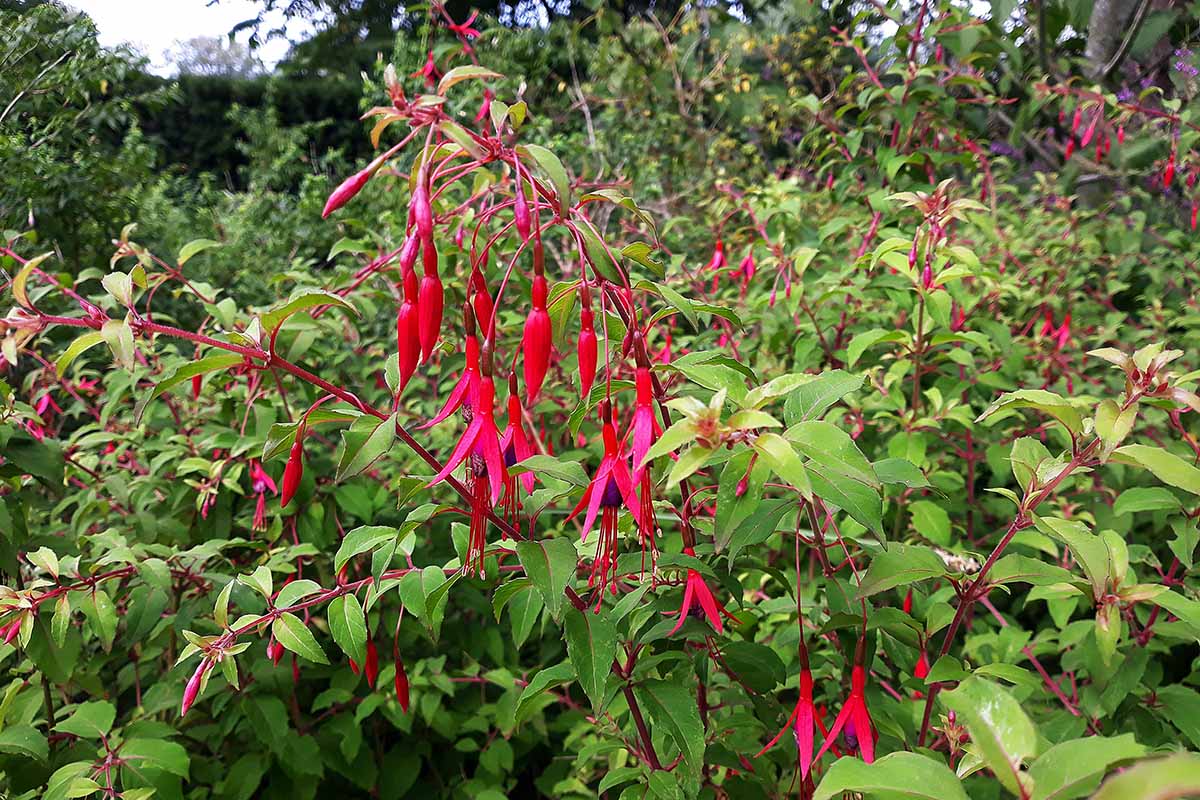
They then made their way to Oregon, Washington, Florida, and Hawaii, primarily infesting plants growing in cool, coastal areas.
The mites bounced across the globe to Europe after likely hitching a ride on plants and were first identified in France in 2002.
In 2007, growers were devastated to learn that the pests had arrived in mainland UK.
These days, wherever fuchsias thrive in South or North America, or Europe, gall mites are sure to be found.
As the pests feed on the plant’s cells, they secrete a poison, which causes new growth to be distorted and misshapen.
Look for disfigured growth tips, flowers, and leaves, as well as galls. Hairy growth is also common.
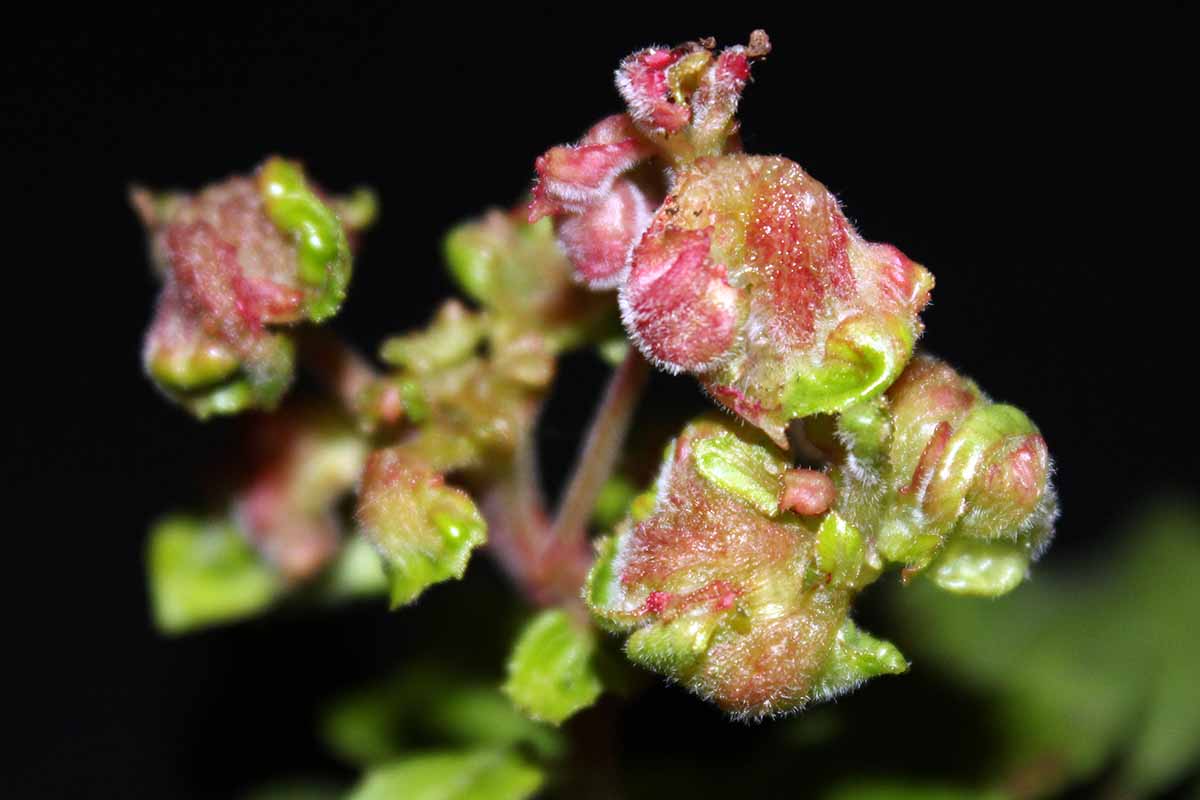
An infestation of aphids can also cause disfigured growth, so don’t immediately assume the presence of twisted leaves, gnarled stems, or stunted tips is a sure sign of mites.
Other symptoms include red or silvery-white discoloration on the stems and foliage. Western flower thrips ( Frankliniella occidentallis ) can also cause distorted, crinkled leaves and silvery coloration that can be confused with the symptoms of gall mites.
The presence of swollen galls on the stems is a more reliable indicator of the presence of these pests
But if you see any of these symptoms, I’d recommend you take a cutting of symptomatic plant tissue, place it in a sealed bag, and show it to your local extension office or fuchsia society.
Experts there can confirm the presence of mites.
Before you bring any cutting or new plant into your garden or home, examine it thoroughly for any signs of pests and quarantine it for at least a month to watch for developing symptoms. Only buy from reputable sources, if you can.
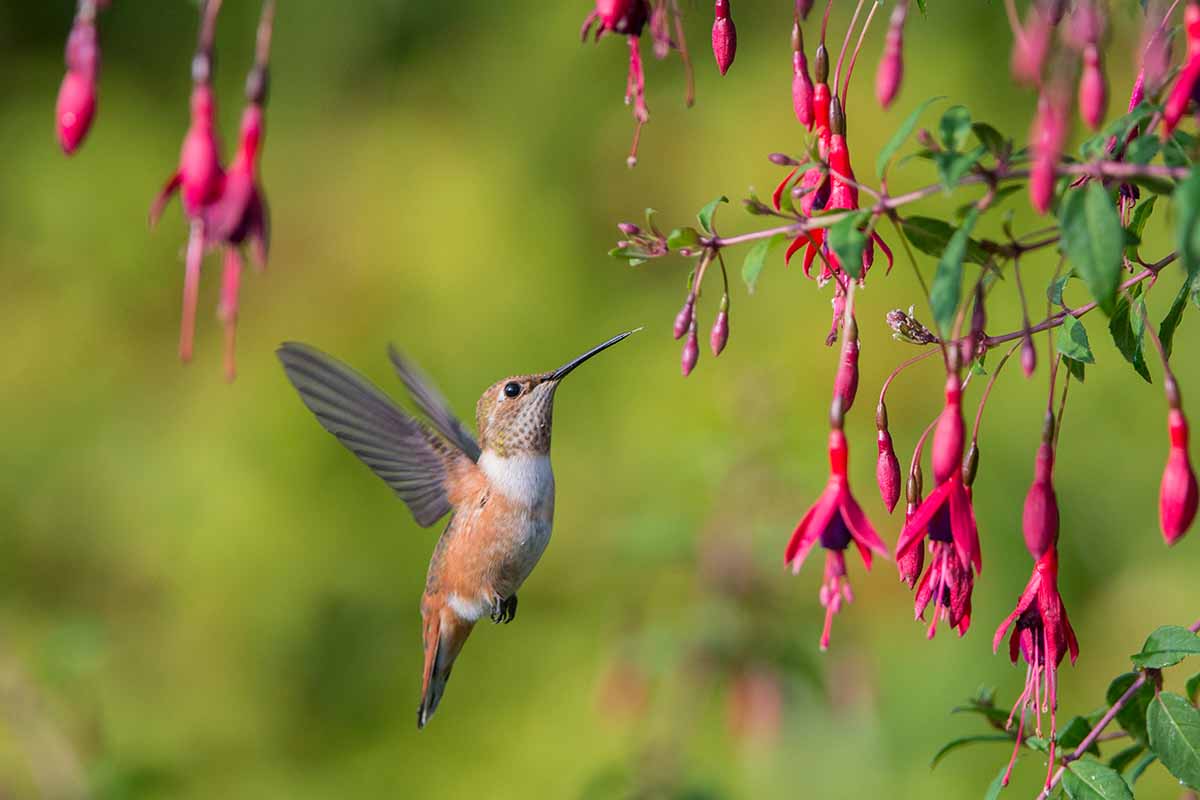
Since the mites can travel on hummingbirds, bees, butterflies, pests, or gardening tools, even these steps don’t guarantee your plants will be safe.
The pests might find their way to your fuchsias.
Always, always clean your tools between uses to be extra safe.
You can wipe your tools in isopropyl alcohol, a 10 percent bleach mixture, or clean them in hot, soapy water to remove any hitchhikers.
Speaking of tools, use your pruners in the early spring or fall to trim woody fuchsia species back to bare wood and tender species back to the ground. Spray the remaining plant material with dormant oil to smother any exposed mites.
Brazilian Fuchsia species have developed some immunity, so consider growing these if you have had trouble with gall mites in the past. F. magellanica , M. coccinea , F. bracelinae , F. regia , F. campos-portoi , F. glazioviana , and F. alpestris are all good options.
Keep in mind that F. magellanica cultivars have been bred outside of Brazil for decades, so only those that come from Brazil in the past few decades will have built up immunity in their native region.
F. splendens , F. paniculata , and F. brevilobis are immune.
The Northwest Fuchsia Society lists ‘Carnival,’ ‘First Success,’ Lechlade Gorgon,’ ‘President Walter Mario,’ ‘Space Shuttle,’ and ‘Tangerine’ as immune.
‘Baby Two Step,’ ‘Bell Buoy,’ ‘Chickadee,’ ‘Golden Anne,’ ‘Jamboree,’ ‘Lena,’ ‘Miracle,’ ‘Mood Indigo,’ ‘Pink Jade,’ ‘Purple Rain,’ ‘Southgate,’ and ‘White King’ are resistant.
Just because a species or cultivar is resistant and immune doesn’t mean that the mites won’t feed on your plants, it’s just that the plants won’t become symptomatic.
Your plants can still be infested and you’ll still need to diligently watch for symptoms on other fuchsias nearby.
As of yet, there isn’t a chemical control that works reliably to eliminate these pests.
The best method of control is to prune off any symptomatic branches and dispose of those in the garbage.
Prune back herbaceous fuchsias to the ground and heavily prune woody plants to bare wood in the fall.
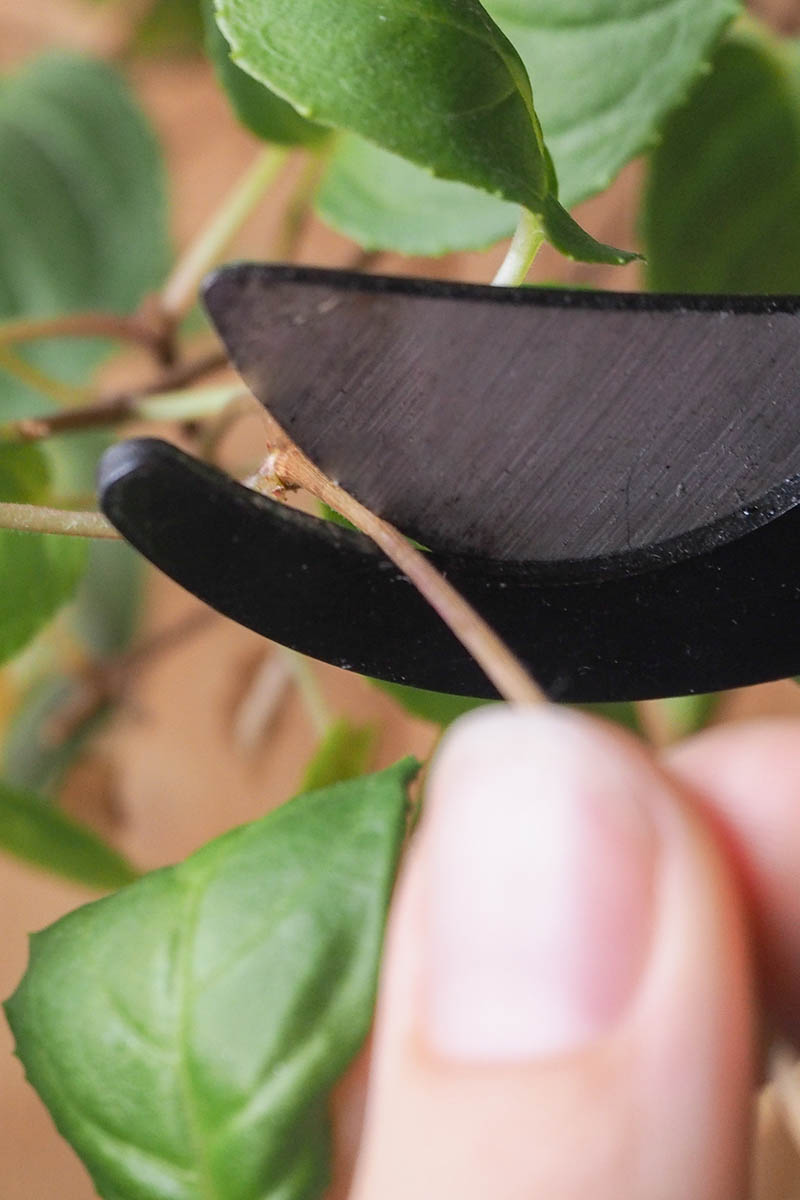
Keep up on this pruning routine as long as the plant shows symptoms.
If you have a plant that is heavily symptomatic, it might be best to toss it in the garbage. Don’t compost infested plant material. If you want to try and save the plant, isolate it from other fuchsias while you work.
To control the infection, trim off all symptomatic parts and wait until the winter when the plant is dormant.
Spray it with a product that contains carbaryl, a dormant oil, or an oil that targets mites such as Bonide’s Mite-X.
Completely saturate the plant to kill all the mites and avoid allowing some to survive and build up immunity to the product.
Spray again after seven days and then again in another seven days. Repeat as long as the plant shows no signs of damage from the spray, such as yellowing leaves or wilting.
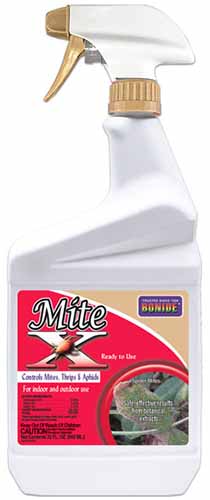
Bonide Mite-X
You can find Bonide Mite-X at Arbico Organics in 12-ounce or quart-sized ready-to-use containers.
Frequent spraying and heavy saturation is vital, because these pests hide in protected spots on the plant where chemical sprays often can’t reach them.
Keep the plant isolated and monitor it through the next growing season. You need to start your spraying routine again at the first sign of symptoms.
If your neighbor has untreated plants, it’s highly likely that the fuchsia gall mites will find those and eventually end up back on your plant at some point. In all honesty, if you have mites in your area, the chances are extremely high that your plants will be infested at some point.
Now, if you want to ensure your plants survive, pruning and spraying is the way to go, but I like to play with fire. Or, rather, ice – cold weather kills these pests.
If tender fuchsias are exposed to extremely cold temperatures, they will die. If the mites are exposed to temperatures around 21°F for a few days, they die.
This temperature is generally considered far too low for tender types, though most hardy fuchsia varieties are just fine.
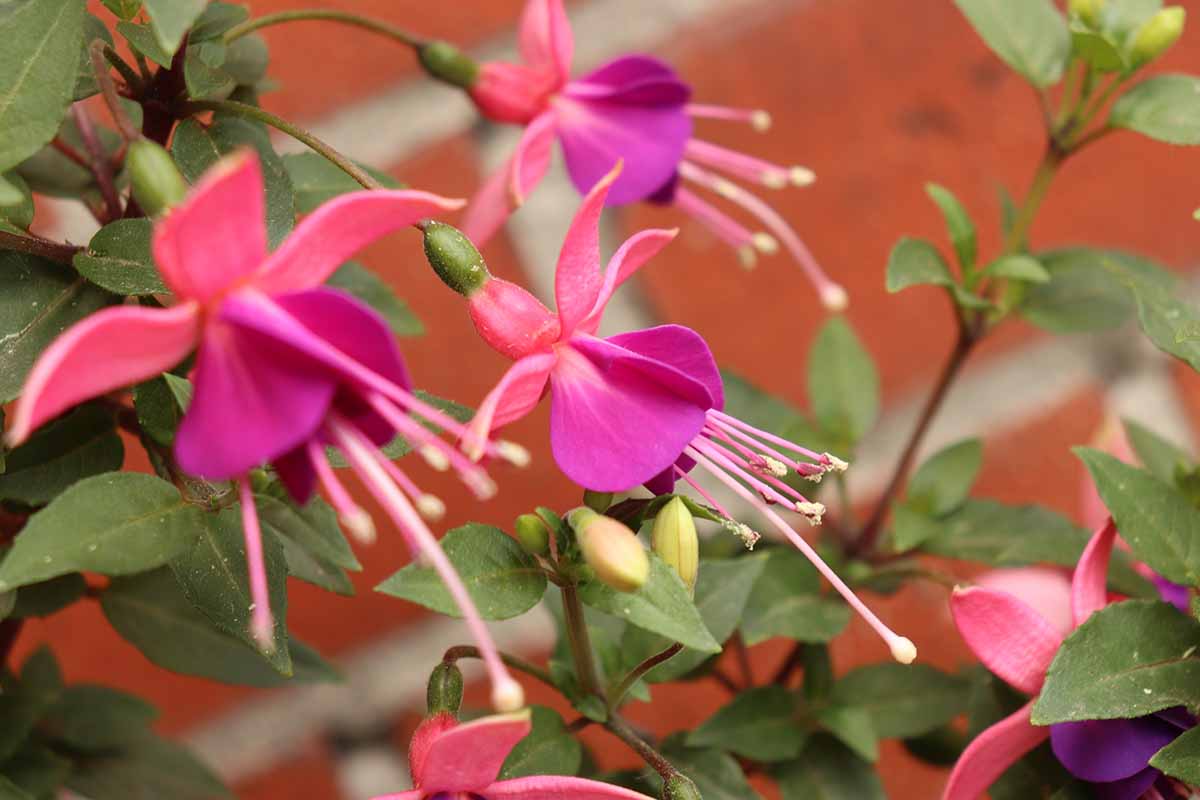
I have left my infested tender fuchsias out in these temperatures figuring I have nothing to lose since they’re already infested. I lost one plant, but the other three survived and haven’t shown symptoms since.
There’s precedence for this method of control. After a few warm winters in the Pacific Northwest, fuchsia plants were being widely infected as of 2005.
But in 2006, there was an extremely cold winter, and those previously infested plants no longer had any damage during the next growing season.
If you can, leave your hardy fuchsias outdoors and let the cold weather kill off the pests.
You can also gamble like I do with your tender fuchsias, but just be aware that the chances are high that you’ll lose them, depending on the species or specific cultivar or hybrid.
But you might lose them to mites, anyway, if they don’t respond to treatment. It’s worth a try if you’re planning to throwing the plant out anyway.
At the very least, we now know that a series of cold winters will greatly reduce gall mite populations, while a few years of warm winters will cause populations to rebound.
While they haven’t yet been proven useful in the field, predaceous mites like Amblyseius californicus show promise in helping to control these pests.
Knowledge is Mightier Than Fuchsia Gall Mites
Fuchsia gall mites are here to stay, at least until some genius scientist comes up with a way to control them.
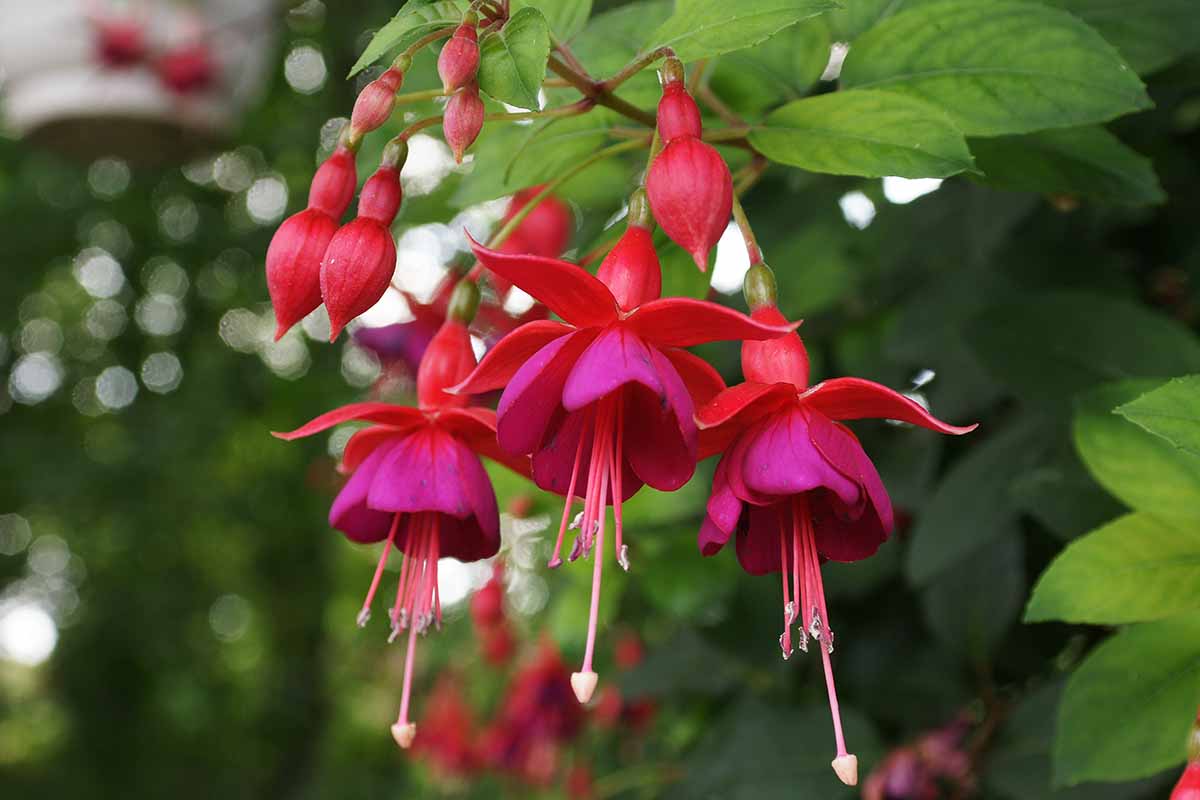
Until then, knowledge is power. Knowing what to watch for and how to take action if mites do arrive will make the difference between losing your plants and them living to fight another day.
Have you dealt with gall mites before? Or do you suspect that they’ve made their way into your garden? Let us know what you’re going through and if we can help with identification or control in the comments section below.
I truly hope this guide helped you avoid trouble or bring your plants back to health. If so, you might want more information about how to care for fuchsias . Check these out next:
- How to Grow Fabulous Fuchsia Flowers
- Are Fuchsia Plants Perennials or Annuals?
- Why Is My Fuchsia Dropping Leaves? 7 Common Issues

Kristine Lofgren
Wait! We have more!
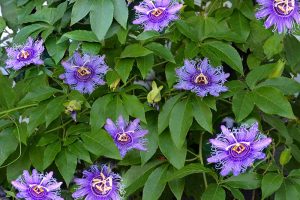
Fertilize Your Passionflower Vines to Boost Growth and Production
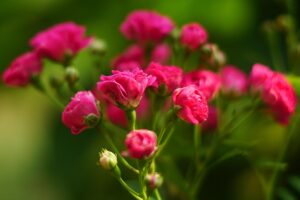
Miniature and Miniflora Roses: What’s the Difference?
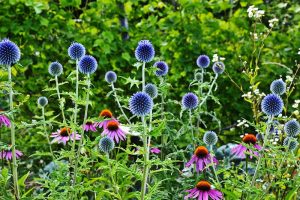
How to Grow and Care for Small Globe Thistle

Dangerous Pests That'll Destroy Your Gardenia (And Tricks To Stop Them)
G ardenia's satiny white aromatic inflorescence has many wrapped around its petals. Unfortunately, like gardeners, pests find them irresistible, harboring in the flowers and foliage every chance they get, damaging and destroying them. Aphids, particularly their melon (Aphis gossypii) and green peach (Myzus persicae) varieties, gorge on these ornamentals since they prefer young, sweet flowers. While they aren't concerning in small clusters, the problem balloons when they multiply over time. Around spring, these tiny, tear-shaped, yellowish-green insects puncture new leaves with their filiform mouths to drink the sap, cutting the foliage's growth short and leaving it disfigured or wilted. They also house various plant viruses.
When extremely severe, these plant lice force the fruits to ripen prematurely and lace the reduced output in their discarded skins and honeydew. As ants find the sugary substance attractive, they jump on the new blooms. This lends the aphids another defense layer while leaving the gardeners with a headache. Another taker of the aphid's dew residue, sooty mold fungi worsens matters and leaves a black, velvety film. The lone green side to the equation is that the fungi don't kill the plant tissue and merely leave the gardenias unsightly. But if they're left on too long, they'll inhibit photosynthesis, predisposing the plant to other diseases and pests.
Read more: How To Get Rid Of Weeds In Your Garden
Whiteflies, Scales, Thrips, And Spider Mites Are Common Culprits
Sooty mold isn't just an aphid accompaniment and features in white flies (Dialeurodes citri) but also scale insects' infestations because they produce honeydew, too. The damage is similar, with the foliage bronzing and dying untimely. But if you notice milky white, moth-like creatures scuttering away on the undersides on shaking the infected plants, know citrus whiteflies are in action. However, an early infestation appears as unmoving, 1.5-millimeters long, light-green scales on the leaves' bases.
In contrast, if relatively bigger — around 6 millimeters wide — white globules are noticeable on the ornamental's stems and shoots, they're most likely a sign of Japanese wax scales (Ceroplastes japonicus) rather than a gardenia disease. But if the blobs appear like elongated egg sacs, then cotton cushion scales (Icerya purchasi) are at play. After sapping all juices, these soft-bodied pests cause leaf drop, branch kill, and poor flowering.
Additionally, flower thrips (Frankliniella tritici) are harder to find because of their microscopic size. Their presence is felt in flowers that prematurely transform into unappealing, malformed browns, withering swiftly—assuming the buds bloom. Moreover, you may notice reddish-purple spots on the curled leaves' bottoms. Conversely, if the spots appear yellowish-white and show signs of webbing, your gardenia may be attacked by a twospotted spider mite (Tetranychus urticae). They'll soon cover your shrubbery in webs, making it look unseemly. The new foliage will grow disfigured.
Dealing With The Pest Problem
Luckily, you can employ multiple ways to nip the pesky problem in the bud. If you catch the aphids early on, simply wash them off with water and repeat the process as necessary. Similarly, remove the infested branches to get rid of scales on plants . If the problem escalates, use biological control. Ladybugs, green lacewings, and parasitic wasps naturally take care of all gardenia pests. However, they don't overwinter and must be reintroduced when spring sets in. Get rid of the ant accomplices by placing baits along their garden trails between the gardenia plants. Alternatively, wrap the shrubbery with fabric or duct tape and coat it with sticky substances to capture the roaming ants. Mix the material every week to prevent debris buildup.
When the pest problem becomes acute, affecting almost 80 to 90% of the leaves, opt for eco-friendly, insecticidal soaps and sprays or horticultural oils. For the highest impact, soak your woody plants deeply to ensure insect contact. Carry out the activity only when the temperatures are under 90 degrees Fahrenheit and the chances of rain the next day are minimal. As these foliar treatments can burn the foliage, use them in the evening after watering the gardenias deeply the day before. Reapply the insecticide at least three times at a gap of five to seven days. For long-lasting results, apply slow-release dinotefuran or imidacloprid insecticides to the soil before the growth season.
Read the original article on House Digest .
- Search Menu
- Sign in through your institution
- Advance Articles
- Special Collections
- Author Guidelines
- Submission Site
- Open Access Options
- Self-Archiving Policy
- Reasons to Submit
- ESA Call for Papers
- Benefits of Publishing Open Access
- About Journal of Economic Entomology
- About the Entomological Society of America
- Editorial Board
- Advertising and Corporate Services
- Dispatch Dates
- Journals on Oxford Academic
- Books on Oxford Academic
Article Contents
- Introduction
- Materials and Methods
- Author Contributions
Neoseiulus mites as biological control agents against Megalurothrips usitatus (Thysanoptera: Thripidae) and Frankliniella intonsa (Thysanoptera: Thripidae) on cowpea crop: laboratory to field

- Article contents
- Figures & tables
- Supplementary Data
Yu-Fei Zhang, Lian-Sheng Zang, Ling-Hang Guo, Singh Sukhwinder, Sheng-Yong Wu, Xiangbing Yang, Liang-De Tang, Neoseiulus mites as biological control agents against Megalurothrips usitatus (Thysanoptera: Thripidae) and Frankliniella intonsa (Thysanoptera: Thripidae) on cowpea crop: laboratory to field, Journal of Economic Entomology , 2024;, toae118, https://doi.org/10.1093/jee/toae118
- Permissions Icon Permissions
Megalurothrips usitatus (Bagnall) (Thysanoptera: Thripidae) and Frankliniella intonsa (Trybom) (Thysanoptera: Thripidae) have been detrimental to cowpea production in many countries. Laboratory experiments were conducted to determine the prey stage preference and functional response of 2 predatory mites species, Neoseiulus barkeri (Hughes) (Acari: Phytoseiidae), and Neoseiulus californicus (McGregor) (Acari: Phytoseiidae), towards 2 thrips species (TS), M. usitatus , and F. intonsa , at varying densities and life stages on cowpea. Results shown that Neoseiulus species had a preference for different life stages of prey. Neoseiulus barkeri consumed more M. usitatus nymphs, while N. californicus consumed more F. intonsa (second-instar nymphs). The functional response of the 2 Neoseiulus spp. to nymphs of 2 TS was Type II on cowpea. The higher attack rate coefficient ( a ʹ) and shorter handling time ( T h ) values were found on N. barkeri against M. usitatus , and a similar trend was found for those in N. californicus against F. intonsa. Field-caged trials were conducted to evaluate the effectiveness of Neoseiulus spp. in controlling 2 TS. The results have shown that Neoseiulus spp. was effective in controlling the 2 TS, with varying control efficacies at high or low release rates. The study provided valuable information on using Neoseiulus spp. as biological control agents against M. usitatus and F. intonsa in cowpea crops.
Email alerts
Citing articles via.
- Entomology Today
- Recommend to Your Librarian
Affiliations
- Online ISSN 1938-291X
- Print ISSN 0022-0493
- Copyright © 2024 Entomological Society of America
- About Oxford Academic
- Publish journals with us
- University press partners
- What we publish
- New features
- Open access
- Institutional account management
- Rights and permissions
- Get help with access
- Accessibility
- Advertising
- Media enquiries
- Oxford University Press
- Oxford Languages
- University of Oxford
Oxford University Press is a department of the University of Oxford. It furthers the University's objective of excellence in research, scholarship, and education by publishing worldwide
- Copyright © 2024 Oxford University Press
- Cookie settings
- Cookie policy
- Privacy policy
- Legal notice
This Feature Is Available To Subscribers Only
Sign In or Create an Account
This PDF is available to Subscribers Only
For full access to this pdf, sign in to an existing account, or purchase an annual subscription.
- LA PRENSA USA
- ESPECTÁCULOS
Baja producción, plagas y hongos afectan la agricultura
- 26 mayo 2024 /
- Redacción Autor marcado para seguir
Productores observan más plantaciones de café con roya, cultivos de papa atacados por la paratrioza y milpas y frijolares por el pulgón amarillo y ácaros.
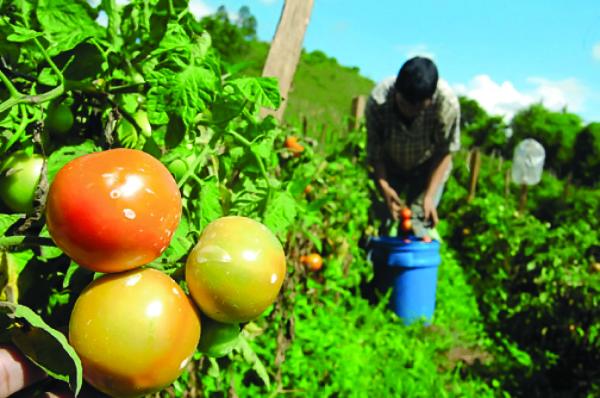
La producción en Honduras está en peligro por las plagas, que polulan por el cambio climático. Foto: LA PRENSA.
Baja producción, plagas, hongos y enfermedades golpean y destruyen parte de las plantaciones agrícolas de Honduras a causa de la ausencia de lluvias y altas temperaturas exacerbadas por el calentamiento global.
Este fenómeno, que es parte del cambio climático global , está generando en el campo condiciones que propician la proliferación de enfermedades e insectos que ponen en jaque la canasta básica de alimentos y la economía hondureña.
En San Francisco de Opalaca , Juan Vásquez está alarmado porque el 80% de las fincas de café de este municipio de Intibucá es afectado por la roya, una de las enfermedades más devastadoras de este sector, que le inyecta a la economía hondureña más de $1,400 millones por las exportaciones.
“Esto es causa del calentamiento global. La roya es una enfermedad que está en la caficultura, pero entre el año pasado y este año nos ha atacado más. Lo que está ocurriendo en las fincas no es normal. Yo tengo seis manzanas y en esta cosecha que pasó saqué algunos 80 galones de café en pergamino, normalmente sacó hasta 800 galones”, dijo Vásquez.
La roya es provocada por el hongo patógeno “ Hemileia vastatrix ”, el cual se propaga a través del viento por medio de las esporas del follaje. Las plantas afectadas por esta enfermedad presentan manchas amarillas, posteriormente marrón oscuro, hasta que se vuelven negras.
“En estos días fumigué la finca, le puse abono. Espero recuperarla, si no me tocará cortar todas las plantas y hacer una nueva finca. Por no tener lluvias seguidas, la tierra está seca y las plantas débiles, por eso son atacadas por los hongos y enfermedades con mayor facilidad”, expresó Vásquez.
En Intibucá, Marlon Martínez, regidor de este municipio, registra una caída del 50% en la producción de papas en sus plantaciones por carecer de fuentes de agua por ausencia de suficientes lluvias en esta región, que comúnmente goza de temperaturas más bajas.
“El rendimiento ha bajado por falta de agua. Cualquier cultivo, si no tiene agua, se ve afectado. En condiciones clave podemos producir hasta 500 quintales por manzana, actualmente, por no tener agua, unos 250 quintales: bajó a la mitad”, manifestó.
Martínez cree que la situación que están viviendo “los productores de papa que se confiaron, los que no esperaban esta sequía, no es normal. Quienes han tenido nacimientos de agua antes regaban los cultivos dos veces por semana, ahora lo hacen una vez por semana”.
La escasez de agua no solo ha provocado un rendimiento de los cultivos de papa, también ha debilitado las plantas, volviéndolas más susceptibles a los ataques de paratrioza (“Paratrioza cockerelli”), un insecto que se desarrolla en diversas condiciones de clima, pero las altas temperaturas aumentan su capacidad de reproducción.
Para enfrentar la paratrioza, según Martínez, “los productores y la Cadena de la Papa capacitarán a todos los que se dedican a este cultivo porque el cambio climático es real y está afectando a todos los agricultores de todo el país”.
Mientras Dulio Medina , presidente de la Asociación de Productores de Granos de Honduras (Prograno), le informó a Diario LA PRENSA que “el pulgón amarillo (‘Rhopalosiphum maidis’) está atacando al maíz y maicillo; los ácaros y trips (Frankliniella) a los frijoles”.
“Estas plagas son difíciles de controlar, solo con insecticidas caros. Necesitamos que el Gobierno abra el mercado de los insecticidas para que entren productos químicos de diferentes marcas y precios, más baratos. Las casas comerciales de químicos están protegidas y acaparan el mercado, no permiten el ingreso de otros productos más baratos y de buena calidad”, dijo.
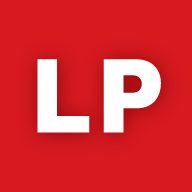
1 Agricultura
2 Café hondureño
3 Cambio climático
6 Producción
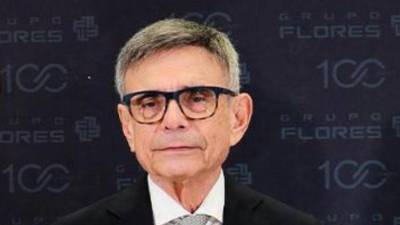
El cuerpo del empresario Alan Flores llega a Medicina Forense
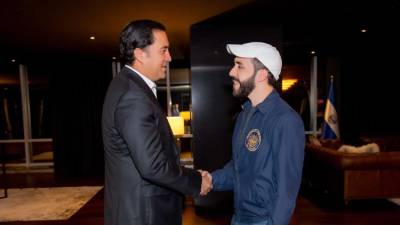
Héctor Zelaya se reúne con el presidente salvadoreño Nayib Bukele
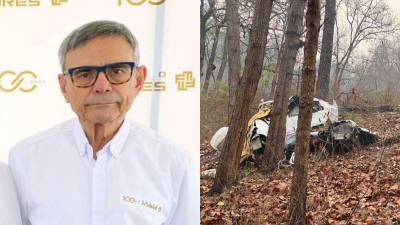
Cohep y CCIC lamentan muerte de empresario Alan Flores en accidente aéreo
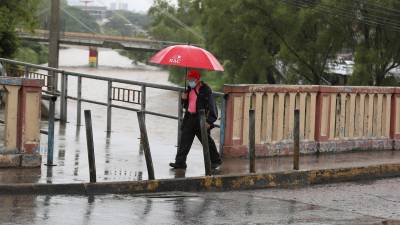
En cuatro regiones habrá lluvias este domingo 26 de mayo en Honduras
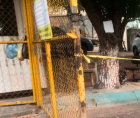
- Más recientes
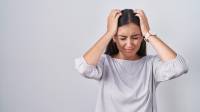
¿Sufre de ansiedad? estos son los mejores ejercicios para mantener la calma
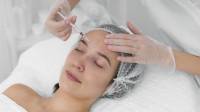
Pros y contras del ácido hialurónico
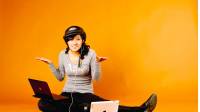
Pubertad precoz: “¿Mi hija es niña o adolescente?”
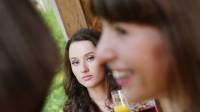
¿Qué hacer cuando la tóxica es tu compañera o amiga?
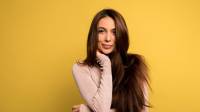
Medidas para proteger el cabello de la contaminación ambiental
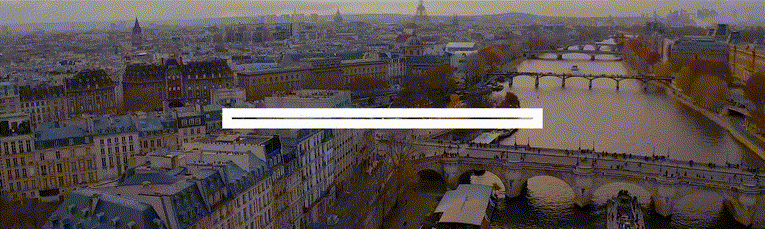
IMAGES
VIDEO
COMMENTS
The western flower thrips [ Frankliniella occidentalis (Pergande)] is an invasive pest insect in agriculture. This species of thrips is native to the Southwestern United States [1] but has spread to other continents, including Europe, Australia (where it was identified in May 1993 [1] ), and South America via transport of infested plant material.
The flower thrips, Frankliniella tritici (Fitch) ( Figure 1) is one of the most abundant species of flower thrips (Thysanoptera: Thripidae) in the eastern United States (Reitz 2008). The official common name established by the Entomological Society of America for this species is flower thrips, although the name flower thrips is often applied ...
Since the 1970s Frankliniella occidentalis has successfully invaded many countries to become one of the most important agricultural pests of ornamental, vegetable and fruit crops globally. Its invasiveness is largely attributed to the international movement of plant material and insecticide resistance, both of which have combined to foster the rapid spread of the species throughout the world ...
Western flower thrips Frankliniella occidentalis. Frankliniella occidentalis (Pergande) (Thysanoptera: Thripidae) originated in western North America and has since become a major pest of vegetables, fruit and ornamental crops across the US and around the world.F. occidentalis are small (1-2 mm long), slender, soft-bodied insects that are yellow to light brown in color; adults have distinctive ...
Management. To sample for Frankliniella thrips or other flower-inhabiting thrips, select a set number of flowers, then either strike these flowers against a light-colored board and count the thrips while still in the field or place the flowers in a vial of ethanol and count the thrips later in the lab (Funderburk et al. 2014a, b). Blue sticky traps may also be used to sample thrips from the field.
Western flower thrips, Frankliniella occidentalis, first arose as an important invasive pest of many crops during the 1970s-1980s. The tremendous growth in international agricultural trade that developed then fostered the invasiveness of western flower thrips. We examine current knowledge regarding the biology of western flower thrips, with an emphasis on characteristics that contribute to ...
The western flower thrips, Frankliniella occidentalis (Pergande), is a globally invasive pest and plant virus vector on a wide array of food, fiber, and ornamental crops. The underlying genetic mechanisms of the processes governing thrips pest and vector biology, feeding behaviors, ecology, and insecticide resistance are largely unknown. To address this gap, we present the F. occidentalis ...
Introduction. Thrips (order Thysanoptera) are minute insects only a few millimeters or less in length. Of the approximately 5500 described species of thrips in the world, scarcely 1% are considered to be serious pests of commercial crops (Morse & Hoddle, 2006; Healey et al., 2017).Among these pests, several species stand out as being among the most important global agricultural pests.
The western flower thrips has established outdoors in areas with milder winters; for example, across the southern U.S.A., southern Europe and Australia. It also overwinters in some regions with colder winters. 6 Polyphagous phytophagous thrips have many factors predisposing them to become worldwide crop pests, particularly in glasshouses.
Western flower thrips (WFT), Frankliniella occidentalis, is one of the most destructive pests of vegetables, fruits and ornamental crops worldwide, causing extensive damage by direct feeding of the crop and transmitting economically important viruses. Despite the successes of biocontrol agents to control WFT, more efficient and cost-effective ways must be found to encourage grower adoption of ...
Introduction. Frankliniella occidentalis (Pergande) (Thysanoptera: Thripidae) originates from North America, began spreading worldwide with the increasing international exchange of horticultural products in the late 1970s, and has become a major greenhouse pest worldwide (Kirk and Terry, 2003).In Korea, F. occidentalis was first found in 1993 on Jeju Island, a far southern island, and spread ...
Frankliniella dianthi Moulton, 1948: 98; Frankliniella syringae Moulton, 1948: 98; Frankliniella umbrosa Moulton, 1948: 105; References. Kirk, WDJ & Terry I. 2003. The spread of western flower thrips Frankliniella occidentalis Pergande. Agricultural and Forest Entomology 5: 301-310. Hoddle MS, Mound LA & Paris D. 2008. Thrips of California.
The flower thrips, Frankliniella intonsa (Trybom) (Thysanoptera: Thripidae), is a small-sized insect pest, well known for feeding and dwelling on the flower of host plants. It is widely ...
Frankliniella insularis (Franklin) Morison (1930) Frankliniella schultzei , the common blossom thrips or cotton thrips , is a species of thrips in the family Thripidae . It is found in many parts of the world and is an important pest insect in agriculture.
Description. Frankliniella occidentalis, the western flower thrips, is a representative of the order Thysanoptera. This insect order is composed exclusively of tiny insects commonly called thrips, and includes more than 7,000 species. Thrips are considered to be members of the hemipteroid assemblage, which includes the orders Hemiptera ...
The flower thrips Frankliniella intonsa (Thysanoptera: Thripidae) is a common insect found in flowers of many plants. Sometimes, F. intonsa causes damage to crops through direct feeding and ...
Thrips feed on a variety of plants including many ornamental, agronomic, vegetable, and wild species. Thrips are very small insects, with adults ranging from 0.5 to 5 mm depending on the species (Jones 2005). Tobacco thrips, Frankliniella fusca (Hinds) ( Figure 1 ), is one of the many species of thrips found throughout Florida. Figure 1.
In the genus Frankliniella, the common blossom thrips, Frankliniella schultzei Trybom, is a relatively new vegetable pest in South Florida. It is a key pest in tomato and cucumber fields in South America. Figure 1. Dorsal view of an adult common blossom thrips, Frankliniella schultzei Trybom. Photograph by Vivek Kumar, Entomology and Nematology ...
Some abiotic and biotic factors affect pepper production under greenhouse conditions. One of the biotic effects is the western flower thrips (WFT), Frankliniella occidentalis (Pergande) (Thysanoptera: Thripidae). The pest attacks many vegetable plants (Kirişik and Erler 2017). F. occidentalis is characterized by its small size, highly reproductive, and spread capability causing a high ...
Pest Common Name. Western flower thrips and onion thrips are the primary damaging thrips species in Idaho. Adult thrips have thin, elongated bodies, feather-like wings and small, dark eyes (Figure 1). Thrips range in color from brown to yellow to cream, depending on the species, sex and life stage. Immature thrips (larvae) are also elongated ...
Abstract New data are presented on two invasive species of thrips in Yakutia: Frankliniella lilivora Kurosawa, 1937, new to the fauna of Russia, and Liothrips vaneeckei Priesner, 1920, new to the fauna of Yakutia. Morphological description of F. lilivora, specific to lily bulbs, and a key to the Russian Frankliniella species are provided.
Currently, the five described species of Thripinema have been recovered from twelve species among eight genera of thrips: Thrips physapus L., Aptinothrips rufus Gmelin, Frankliniella vaccinia Morgan, Frankliniella occidentalis Pergande, Frankliniella fusca Hinds, Taeniothrips vaccinophilus Hood, Stenothrips graminium Uzel, Catinathrips ...
Western flower thrips (Frankliniella occidentallis) can also cause distorted, crinkled leaves and silvery coloration that can be confused with the symptoms of gall mites. The presence of swollen galls on the stems is a more reliable indicator of the presence of these pests.
Additionally, flower thrips (Frankliniella tritici) are harder to find because of their microscopic size. Their presence is felt in flowers that prematurely transform into unappealing, malformed ...
Abstract. Megalurothrips usitatus (Bagnall) (Thysanoptera: Thripidae) and Frankliniella intonsa (Trybom) (Thysanoptera: Thripidae) have been detrimental to cowpea production in many countries. Laboratory experiments were conducted to determine the prey stage preference and functional response of 2 predatory mites species, Neoseiulus barkeri (Hughes) (Acari: Phytoseiidae), and Neoseiulus ...
Heliothrips haemorrhoidalis is a species of thrips in the family Thripidae. It is most commonly known as the greenhouse thrips, the glasshouse thrip or black tea thrips. This species of thrips was first described in 1833 by Bouché in Berlin, Germany. H. haemorrhoidalis also has many synonyms depending on where they were described from such as: H. adonidum Haliday, H. semiaureus Girault, H ...
55 likes, 2 comments - raspadacanela on March 22, 2024: "Frankliniella schultzei (trips) 漣漣漣. . @ledsindoor . Octane? @koppert_brasil @koppertcanada @koppert_global".
El clavel o clavelina 1 ( Dianthus caryophyllus) es una planta herbácea perteneciente a la familia de las Caryophyllaceae, es considerada como flor del agua, difundida en las regiones mediterráneas 2 Es espontánea en la flora de la península ibérica. En su forma típica es una planta cespitosa, 2 con numerosos vástagos de hasta 1 m de altura.
Several species of entomopathogenic nematodes in the genus Thripinema are known to naturally parasitize thrips (Thysanoptera). Thripinema fuscum Tipping and Nguyen is the most common species in Florida ( Figure 1 ). Thripinema fuscum is economically important because it is a natural enemy of the insect pest, the tobacco thrips, Frankliniella ...
San Pedro Sula. Baja producción, plagas, hongos y enfermedades golpean y destruyen parte de las plantaciones agrícolas de Honduras a causa de la ausencia de lluvias y altas temperaturas ...